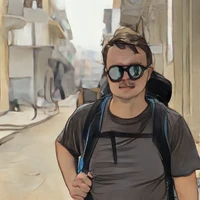
pszemraj/long-t5-tglobal-base-sci-simplify-elife
Summarization
•
Updated
•
413
•
5
article
stringlengths 1.95k
164k
| summary
stringlengths 987
4.09k
| section_headings
stringlengths 13
268
| keywords
stringlengths 0
97
| year
stringclasses 11
values | title
stringlengths 30
189
| article_length
int64 452
51k
| summary_length
int64 242
870
|
---|---|---|---|---|---|---|---|
In temperate climates, winter deaths exceed summer ones. However, there is limited information on the timing and the relative magnitudes of maximum and minimum mortality, by local climate, age group, sex and medical cause of death. We used geo-coded mortality data and wavelets to analyse the seasonality of mortality by age group and sex from 1980 to 2016 in the USA and its subnational climatic regions. Death rates in men and women ≥ 45 years peaked in December to February and were lowest in June to August, driven by cardiorespiratory diseases and injuries. In these ages, percent difference in death rates between peak and minimum months did not vary across climate regions, nor changed from 1980 to 2016. Under five years, seasonality of all-cause mortality largely disappeared after the 1990s. In adolescents and young adults, especially in males, death rates peaked in June/July and were lowest in December/January, driven by injury deaths.
It is well-established that death rates vary throughout the year, and in temperate climates there tend to be more deaths in winter than in summer (Campbell, 2017; Fowler et al. , 2015; Healy, 2003; McKee, 1989). It has therefore been hypothesized that a warmer world may lower winter mortality in temperate climates (Langford and Bentham, 1995; Martens, 1998). In a large country like the USA, which possesses distinct climate regions, the seasonality of mortality may vary geographically, due to geographical variations in mortality, localized weather patterns, and regional differences in adaptation measures such as heating, air conditioning and healthcare (Davis et al. , 2004; Braga et al. , 2001; Kalkstein, 2013; Medina-Ramón and Schwartz, 2007). The presence and extent of seasonal variation in mortality may also itself change over time (Bobb et al. , 2014; Carson et al. , 2006; Seretakis et al. , 1997; Sheridan et al. , 2009). A thorough understanding of the long-term dynamics of seasonality of mortality, and its geographical and demographic patterns, is needed to identify at-risk groups, plan responses at the present time as well as under changing climate conditions. Although mortality seasonality is well-established, there is limited information on how seasonality, including the timing of minimum and maximum mortality, varies by local climate and how these features have changed over time, especially in relation to age group, sex and medical cause of death (Rau, 2004; Rau et al. , 2018). In this paper, we comprehensively characterize the spatial and temporal patterns of all-cause and cause-specific mortality seasonality in the USA by sex and age group, through the application of wavelet analytical techniques, to over three decades of national mortality data. Wavelets have been used to study the dynamics of weather phenomena (Moy et al. , 2002) and infectious diseases (Grenfell et al. , 2001). We also used centre of gravity analysis and circular statistics methods to understand the timing of maximum and minimum mortality. In addition, we identify how the percentage difference between death rates in maximum and minimum mortality months has changed over time.
The strengths of our study are its innovative methods of characterizing seasonality of mortality dynamically over space and time, by age group and cause of death; using wavelet and centre of gravity analyses; using ERA-Interim data output to compare the association between seasonality of death rates and regional temperature. A limitation of our study is that we did not investigate seasonality of mortality by socioeconomic characteristics which may help with understanding its determinants and planning responses.
We used wavelet and centre of gravity analyses, which allowed systematically identifying and characterizing seasonality of total and cause-specific mortality in the USA, and examining how seasonality has changed over time. We identified distinct seasonal patterns in relation to age and sex, including higher all-cause summer mortality in young men (Feinstein, 2002; Rau et al. , 2018). Importantly, we also showed that all-cause and cause-specific mortality seasonality is largely similar in terms of both timing and magnitude across diverse climatic regions with substantially different summer and winter temperatures. Insights of this kind would not have been possible analysing data averaged over time or nationally, or fixed to pre-specified frequencies. Prior studies have noted seasonality of mortality for all-cause mortality and for specific causes of death in the USA (Feinstein, 2002; Kalkstein, 2013; Rau, 2004; Rau et al. , 2018; Rosenwaike, 1966; Seretakis et al. , 1997). Few of these studies have done consistent national and subnational analyses, and none has done so over time, for a comprehensive set of age groups and causes of death, and in relation to regional temperature differences. Our results on strong seasonality of cardiorespiratory diseases deaths and weak seasonality of cancer deaths, restricted to older ages, are broadly consistent with these studies (Feinstein, 2002; Rau et al. , 2018; Rosenwaike, 1966; Seretakis et al. , 1997), which had limited analysis on how seasonality changes over time and geography (Feinstein, 2002; Rau et al. , 2018; Rosenwaike, 1966). Similarly, our results on seasonality of injury deaths are supported by a few prior studies (Feinstein, 2002; Rau et al. , 2018; Rosenwaike, 1966), but our subnational analysis over three decades revealed variations in when injury deaths peaked and in how seasonal differences in these deaths have changed over time in relation to age group which had not been reported before. A study of 36 cities in the USA, aggregated across age groups and over time, also found that excess mortality was not associated with seasonal temperature range (Kinney et al. , 2015). In contrast, a European study found that the difference between winter and summer mortality was lower in colder Nordic countries than in warmer southern European nations (Healy, 2003; McKee, 1989) (the study’s measure of temperature was mean annual temperature which differed from the temperature difference between maximum and minimum mortality used in our analysis although the two measures are correlated). The absence of variation in the magnitude of mortality seasonality indicates that different regions in the USA are similarly adapted to temperature seasonality, whereas Nordic countries may have better environmental (e. g. housing insulation and heating) and health system measures to counter the effects of cold winters than those in southern Europe. If the observed absence of association between the magnitude of mortality seasonality and seasonal temperature difference across the climate regions also persists over time, the changes in temperature as a result of global climate change are unlikely to affect the winter-summer mortality difference. The cause-specific analysis showed that the substantial decline in seasonal mortality differences in adolescents and young adults was related to the diminishing seasonality of (unintentional) injuries, especially from road traffic crashes, which are more likely to occur in the summer months (Liu et al. , 2005) and are more common in men. The weakening of seasonality in boys under five years of age was related to two phenomena: first, the seasonality of death from cardiorespiratory diseases declined, and second, the proportion of deaths from perinatal conditions, which exhibit limited seasonality (Figure 9—figure supplement 2 and Figure 10—figure supplement 3), increased (MacDorman and Gregory, 2015). In contrast to young and middle ages, mortality in older ages, where death rates are highest, maintained persistent seasonality over a period of three decades (we note that although the percent seasonal difference in mortality has remained largely unchanged in these ages, the absolute difference in death rates between the peak and minimum months has declined because total mortality has a declining long-term trend). This finding demonstrates the need for environmental and health service interventions targeted towards this group irrespective of geography and local climate. Examples of such interventions include enhancing the availability of both environmental and medical protective factors, such as better insulation of homes, winter heating provision and flu vaccinations, for the vulnerable older population (Katiyo et al. , 2017). Social interventions, including regular visits to the isolated elderly during peak mortality periods to ensure that they are optimally prepared for adverse conditions, and responsive and high-quality emergency care, are also important to protect this vulnerable group (Healy, 2003; Lerchl, 1998; Katiyo et al. , 2017). Emergent new technologies, such as always-connected hands-free communications devices with the outside world, in-house cameras, and personal sensors also provide an opportunity to enhance care for the older, more vulnerable groups in the population, especially in winter when the elderly have fewer social interactions (Morris, 2013). Such interventions are important today, and will remain so as the population ages and climate change increases the within- and between-season weather variability.
We used data on all 85,854,176 deaths in the USA from 1980 to 2016 from the National Center for Health Statistics (NCHS). Age, sex, state of residence, month of death, and underlying cause of death were available for each record. The underlying cause of death was coded according to the international classification of diseases (ICD) system (9th revision of ICD from 1980 to 1998 and 10th revision of ICD thereafter). Yearly population counts were available from NCHS for 1990 to 2016 and from the US Census Bureau prior to 1990 (Ingram et al. , 2003). We calculated monthly population counts through linear interpolation, assigning each yearly count to July. We also subdivided the national data geographically into nine climate regions used by the National Oceanic and Atmospheric Administration (Figure 18 and Table 2) (Karl and Koss, 1984). On average, the Southeast and South are the hottest climate regions with average annual temperatures of 18. 4°C and 18°C respectively; the South also possesses the highest average maximum monthly temperature (27. 9°C in July). The lowest variation in temperature throughout the year is that of the Southeast (an average range of 17. 5°C). The three coldest climate regions are West North Central, East North Central and the Northwest (7. 6°C, 8. 0°C, 8. 2°C respectively). Mirroring the characteristics of the hottest climate regions, the largest variation in temperature throughout the year is that of the coldest region, West North Central (an average range of 30. 5°C), which also has the lowest average minimum monthly temperature (−6. 5°C in January). The other climate regions, Northeast, Southwest, and Central, possess similar average temperatures (10°C to 14°C) and variation within the year of (23°C to 26°C), with the Northeast being the most populous region in the United States (with 19. 8% total population in 2016). Data were divided by sex and age in the following 10 age groups: 0–4,5–14,15-24,25–34,35–44,45–54,55–64,65–74,75–84,85+ years. We calculated monthly death rates for each age and sex group, both nationally and for sub-national climate regions. Death rate calculations accounted for varying length of months, by multiplying each month’s death count by a factor that would make it equivalent to a 31 day month. For analysis of seasonality by cause of death, we mapped each ICD-9 and ICD-10 codes to four main disease categories (Table 1) and to a number of subcategories which are presented in the Supplementary Note. Cardiorespiratory diseases and cancers accounted for 56. 4% and 21. 2% of all deaths in the USA, respectively, in 1980, and 40. 3% and 22. 4%, respectively, in 2016. Deaths from cardiorespiratory diseases have been associated with cold and warm temperatures (Basu, 2009; Basu and Samet, 2002; Bennett et al. , 2014; Braga et al. , 2002; Gasparrini et al. , 2015). Injuries, which accounted for 8% of all deaths in the USA in 1980 and 7. 3% in 2016, may have seasonality that is distinct from so-called natural causes. We did not further divide other causes because the number of deaths could become too small to allow stable estimates when divided by age group, sex and climate region. We obtained data on temperature from ERA-Interim, which combines predictions from a physical model with ground-based and satellite measurements (Dee et al. , 2011). We used gridded four-times-daily estimates at a resolution of 80 km to generate monthly population-weighted temperature by climate region throughout the analysis period. We used wavelet analysis to investigate seasonality for each age-sex group. Wavelet analysis uncovers the presence, and frequency, of repeated maxima and minima in each age-sex-specific death rate time series (Hubbard, 1998; Torrence and Compo, 1998). In brief, a Morlet wavelet, described in detail elsewhere (Cazelles et al. , 2008), is equivalent to using a moving window on the death rate time series and analysing periodicity in each window using a short-form Fourier transform, hence generating a dynamic spectral analysis, which allows measuring dynamic seasonal patterns, in which the periodicity of death rates may disappear, emerge, or change over time. In addition to coefficients that measure the frequency of periodicity, wavelet analysis estimates the probability of whether the data are different from the null situation of random fluctuations that can be represented with white (an independent random process) or red (autoregressive of order one process) noise. For each age-sex group, we calculated the p-values of the presence of 12 month seasonality for the comparison of wavelet power spectra of the entire study period (1980–2016) with 100 simulations against a white noise spectrum, which represents random fluctuations. We used the R package WaveletComp (version 1. 0) for the wavelet analysis. Before analysis, we de-trended death rates using a polynomial regression, and rescaled each death rate time series so as to range between 1 and −1. To identify the months of maximum and minimum death rates, we calculated the centre of gravity and the negative centre of gravity of monthly death rates. Centre of gravity was calculated as a weighted average of months of deaths, with each month weighted by its death rate; negative centre of gravity was also calculated as a weighted average of months of deaths, but with each month was weighted by the difference between its death rate and the year’s maximum death rate. In taking the weighted average, we allowed December (month 12) to neighbour January (month 1), representing each month by an angle subtended from 12 equally-spaced points around a unit circle. Using a technique called circular statistics, a mean (θ-) of the angles (θ1, θ2, θ3…, θn,) representing the deaths (with n the total number of deaths in an age-sex group for a particular cause of death) is found using the relation below: θ-=arg∑j=1nexp (iθj), where arg denotes the complex number argument and θj denotes the month of death in angular form for a particular death j. The outcome of this calculation is then converted back into a month value (Fisher, 1995). Along with each circular mean, a 95% confidence interval (CI) was calculated by using 1000 bootstrap samples. The R package CircStats (version 0. 2. 4) was used for this analysis. For each age-sex group and cause of death, and for each year, we calculated the percent difference in death rates between the maximum and minimum mortality months. We fitted a linear regression to the time series of seasonal differences from 1980 to 2016, and used the fitted trend line to estimate how much the percentage difference in death rates between the maximum and minimum mortality months had changed from 1980 to 2016. We weighted seasonal difference by the inverse of the square of its standard error, which was calculated using a Poisson model to take population size of each age-sex group through time into account. This method gives us a p-value for the change in seasonal difference per year, which we used to calculate the seasonal difference at the start (1980) and end (2016) of the period of study. Our method of analysing seasonal differences avoids assuming that any specific month or group of months represent highest and lowest number of deaths for a particular cause of death, which is the approach taken by the traditional measure of Excess Winter Deaths. It also allows the maximum and minimum mortality months to vary by age group, sex and cause of death. | In the USA, more deaths happen in the winter than the summer. But when deaths occur varies greatly by sex, age, cause of death, and possibly region. Seasonal differences in death rates can change over time due to changes in factors that cause disease or affect treatment. Analyzing the seasonality of deaths can help scientists determine whether interventions to minimize deaths during a certain time of year are needed, or whether existing ones are effective. Scrutinizing seasonal patterns in death over time can also help scientists determine whether large-scale weather or climate changes are affecting the seasonality of death. Now, Parks et al. show that there are age and sex differences in which times of year most deaths occur. Parks et al. analyzed data on US deaths between 1980 and 2016. While overall deaths in a year were highest in winter and lowest in summer, a greater number of young men died during summer – mainly due to injuries – than during winter. Seasonal differences in deaths among young children have largely disappeared and seasonal differences in the deaths of older children and young adults have become smaller. Deaths among women and men aged 45 or older peaked between December and February – largely caused by respiratory and heart diseases, or injuries. Deaths in this older age group were lowest during the summer months. Death patterns in older people changed little over time. No regional differences were found in seasonal death patterns, despite large climate variation across the USA. The analysis by Parks et al. suggests public health and medical interventions have been successful in reducing seasonal deaths among many groups. But more needs to be done to address seasonal differences in deaths among older adults. For example, by boosting flu vaccination rates, providing warnings about severe weather and better insulation for homes. Using technology like hands-free communication devices or home visits to help keep vulnerable elderly people connected during the winter months may also help. | Abstract
Introduction
Results
Discussion
Materials and methods | epidemiology and global health | 2018 | National and regional seasonal dynamics of all-cause and cause-specific mortality in the USA from 1980 to 2016 | 3,749 | 403 |
Whether complement dysregulation directly contributes to the pathogenesis of peripheral nervous system diseases, including sensory neuropathies, is unclear. We addressed this important question in a mouse model of ocular HSV-1 infection, where sensory nerve damage is a common clinical problem. Through genetic and pharmacologic targeting, we uncovered a central role for C3 in sensory nerve damage at the morphological and functional levels. Interestingly, CD4 T cells were central in facilitating this complement-mediated damage. This same C3/CD4 T cell axis triggered corneal sensory nerve damage in a mouse model of ocular graft-versus-host disease (GVHD). However, this was not the case in a T-dependent allergic eye disease (AED) model, suggesting that this inflammatory neuroimmune pathology is specific to certain disease etiologies. Collectively, these findings uncover a central role for complement in CD4 T cell-dependent corneal nerve damage in multiple disease settings and indicate the possibility for complement-targeted therapeutics to mitigate sensory neuropathies.
Dysregulated complement activation is increasingly recognized as a significant pathological event in a variety of neurodegenerative and neuroinflammatory diseases of the central nervous system (Tenner et al. , 2018). These include conditions from Alzheimer’s disease and age-related macular degeneration (AMD) to amyotrophic lateral sclerosis and multiple sclerosis (Hong et al. , 2016; Knickelbein et al. , 2015; Lee et al. , 2018; Loveless et al. , 2018). Complement may orchestrate peripheral nervous system (PNS) disorders as well. For instance, nociceptive hypersensitivities, sensory neuropathies, and Guillain-Barré syndrome have been linked to aberrant complement activation (Rosoklija et al. , 2000; Ramaglia et al. , 2008; Jang et al. , 2010; Fritzinger and Benjamin, 2016; Xu et al. , 2018; Susuki et al. , 2007). However, the mechanistic role of complement is not well understood in neuroinflammatory pathologies impacting the PNS. Moreover, the pathophysiological trigger of sensory fiber retraction which contributes to sensation loss and in some instances chronic pain is unclear (Stepp et al. , 2017). Available treatments for peripheral neuropathies primarily focus on clinical symptoms without addressing the underlying pathomechanisms (Colloca et al. , 2017). Accordingly, elucidation of relevant pathomechanisms underlying sensory neuropathies may translate into efficacious mechanism-based therapeutics. The complement cascade is comprised of dozens of soluble and membrane-bound factors essential for host defense and efficient clearance of cellular debris. However, proper regulation of complement activation is necessary to balance immune responses and prevent collateral tissue damage (Ricklin et al. , 2016). The complement cascade pivots upon activation of complement component 3 (C3) for its downstream proinflammatory effects. The pathogenic contributions of complement in systemic disease have been reviewed extensively in recent years (Dobó et al. , 2018; Hajishengallis et al. , 2017; McGeer et al. , 2017; Ricklin et al. , 2016). Nonetheless, emerging concepts centering on complement effector synthesis within inflamed tissue microenvironments have ushered in a ‘renaissance’ of novel complement-targeted drug development strategies (Ricklin et al. , 2018; Tomlinson and Thurman, 2018). Therefore, elucidating whether the complement cascade is a viable therapeutic target in inflammation-associated peripheral nerve impairment remains medically important. The eye has been labeled a ‘complement dysregulation hotspot’ due to complement’s contributions to many ophthalmic diseases, but complement activation is restricted in the healthy cornea (Clark and Bishop, 2018). As observed in AMD, subtle inflammatory reactions in the eye can mediate significant visual morbidity. Accordingly, ocular inflammation is tightly regulated by various anatomic, physiologic, and immunologic mechanisms in order to maintain visual acuity (Amouzegar et al. , 2016; Streilein, 2003; Taylor et al. , 2018). These homeostatic mechanisms, collectively dubbed ‘ocular immune privilege, ’ help preserve the ocular surface and enable the cornea to properly focus incoming light onto the retina. Furthermore, corneal nerves are increasingly recognized as central regulators of immune privilege at the ocular surface (Guzmán et al. , 2018; Neelam et al. , 2018; Paunicka et al. , 2015). Consequently, inflammatory events that damage corneal nerves can have insidious consequences in terms of ocular surface health and transparency (Müller et al. , 2003; Shaheen et al. , 2014; Stepp et al. , 2017). One such pathway that can rapidly initiate inflammation following activation is the complement cascade. Corneal nerves originate predominantly from the ophthalmic branch of the trigeminal ganglion (TG), and these peripheral nerves provide the cornea with the highest density of sensory fibers in the human body. While the cornea and peripheral nerves constitutively synthesize complement proteins (Bora et al. , 2008; de Jonge et al. , 2004), the role of the complement cascade in corneal nerve damage has not been explored. Nonetheless, the complement pathway is poised for vigorous activation in the cornea in response to noxious or inflammatory stimuli (Bora et al. , 2008). Moreover, C3 activation has been reported in the cornea soon after infection with herpes simplex virus type 1 (HSV-1), which is a common cause of corneal sensory nerve damage in patients (Royer et al. , 2017; Sacchetti and Lambiase, 2014). Given the cornea’s high density of sensory nerves, it is particularly amenable for investigating the mechanistic role of complement in peripheral nerve damage. To this end, we evaluated corneal nerve integrity and mechano-sensory function using a murine model of ocular HSV-1 infection to test the hypothesis that local complement activation and T cell engagement coordinate corneal nerve damage. The pathobiology underlying non-penetrating corneal nerve damage is not well understood, although inflammation is generally recognized as an important feature (Neelam et al. , 2018; Shaheen et al. , 2014; Cruzat et al. , 2015; Chucair-Elliott et al. , 2016; Chucair-Elliott et al. , 2017b; Chucair-Elliott et al. , 2017a). While HSV-1 is a clinically prominent cause of corneal nerve damage and sensation loss, a variety of pathogenic microbes impair the corneal nerve architecture upon ocular infection (Cruzat et al. , 2015). This observation adds to evidence that the neurotropism of HSV-1 is not directly responsible for nerve damage (Chucair-Elliott et al. , 2016). In addition to sensation loss evoked by HSV-1, corneal nerve alterations in other contexts such as dry eye disease are associated with a broad array of neuropathic clinical symptoms including dryness, itch, and pain (Andersen et al. , 2017). To further qualify the possible role of complement in corneal nerve damage independent of infection, we evaluated corneal mechanosensory function in two noninfectious T cell-dependent ocular surface inflammatory diseases. For this purpose, we utilized established murine models of allergic eye disease (AED) and ocular graft-versus-host disease (GVHD) (Herretes et al. , 2015; Lee et al. , 2015). Our rationale for this is that complement has been implicated in the etiology of systemic GVHD and allergic inflammation (Gour et al. , 2018; Kwan et al. , 2012; Ma et al. , 2014; Nguyen et al. , 2018; Zhang and Köhl, 2010). However, a neuroinflammatory role of complement has not been described for either disease within the eye. The translational relevance of this study is underscored by in vivo confocal microscopy data from multiple clinical studies showing architectural changes in the corneal nerves of patients with herpetic keratitis, chronic ocular allergy, and ocular GVHD (Hamrah et al. , 2010; Müller et al. , 2015; Moein et al. , 2018; Hu et al. , 2008; Le et al. , 2011; Leonardi et al. , 2012; Tepelus et al. , 2017; He et al. , 2017a). Elucidating the pathomechanisms underlying corneal nerve damage may enable development of more effective therapeutics to mitigate progression of such ocular surface inflammatory diseases. Each of the animal models utilized herein mimic clinically important, chronic ocular surface morbidities. Herpes simplex virus type 1 (HSV-1) is a common cause of neurotropic keratitis and remains a leading cause of infectious corneal blindness (Sacchetti and Lambiase, 2014; Farooq and Shukla, 2012). The incidence of ocular allergy exceeds twenty percent of the population with varying degrees of neurogenic ocular surface discomfort that can severely diminish quality of life (Craig et al. , 2017; Patel et al. , 2017; Saban et al. , 2013). Finally, GVHD is the greatest cause of non-relapse morbidity following hematopoietic stem cell transplantation (HSCT) used to treat life-threatening malignancies and immunologic diseases (MacDonald et al. , 2017). A majority of patients with chronic presentations of GVHD suffer from ocular surface involvement (Shikari et al. , 2013).
Adaptive immunity has been shown to prevent recovery of corneal sensory function following ocular HSV-1 infection (Yun et al. , 2014), but the initial pathophysiological triggers of denervation and sensation loss are not definitively characterized. Ocular HSV-1 infection provokes corneal denervation and sensation loss between days 5 to 8 post-infection (p. i.) in immunologically naive C57BL/6 mice (Chucair-Elliott et al. , 2015). This tempo appears to be synchronized with the host transition from innate to adaptive immunity following infection. Indeed, corneal nerve fiber retraction was evident upon T cell infiltration in the cornea (Figure 1A). Accordingly, we hypothesized that local complement activation and T cell engagement coordinate corneal nerve damage during HSV-1 infection. To address our hypothesis, corneal sensation and pathogen burden were evaluated in C57BL/6 wildtype (WT) and complement C3-deficient (C3-/-) mice following ocular HSV-1 infection. Progressive loss of corneal mechano-sensitivity was evident in WT mice by days 5 to 8 p. i. , but corneal sensation was conserved in the C3-/- cohort (Figure 1B). The same trend revealing preservation of corneal sensation in C3-/- animals was also observed with an increased HSV-1 challenge inoculum (Figure 1—figure supplement 1). Viral titers were measured at time points before and after the onset of corneal sensation loss to determine whether the divergence in sensation stemmed from a differential susceptibility to infection. On day 3 p. i. , the HSV-1 burden was similar in the tear film, corneas, and TG of WT and C3-/- animals (Figure 1C–D). By day 7 p. i. , HSV-1 titers were elevated in the tear film and TG of C3-/- mice relative to WT (Figure 1C, E). Corneal buttons from WT mice exhibited extensive denervation with CD3+ T cell infiltration at day 8 p. i. (Figure 1F). However, T cell infiltration was observed without widespread denervation in C3-/- corneas (Figure 1F). The number of cornea-infiltrating CD4+ or CD8+ T cells were similar among WT and C3-/- mice (Figure 1G). Together, these data indicate that corneal sensation loss in herpetic keratitis involves a C3-dependent inflammatory process independent of viral burden. Although T cells successfully extravasate into the corneas of WT and C3-/- mice (Figure 1F, G), C3-deficiency can have broad impacts on T cell priming, clonal expansion, and recruitment (Clarke and Tenner, 2014; West et al. , 2018). To investigate this possibility, corneas from HSV-1-infected WT and C3-/- mice were evaluated for chemokines associated with T cell recruitment, and the eye-draining mandibular lymph nodes (MLN) were harvested to evaluate T cell responses. Chemokines associated with T cell recruitment were elevated in corneas from both WT and C3-/- mice at day 5 p. i. —a time consistent with onset of sensation loss (Figure 2A). Moreover, CD4+ and CD8+ T cell expansion was comparable within the eye-draining mandibular lymph nodes (MLN) from WT and C3-/- mice (Figure 2B). Similarly, T cells harvested from WT and C3-/- mice at day 8 p. i. responded equally to in vitro stimulation in terms of IFNγ production (Figure 2C). Collectively, these data show that C3-/- T cells exhibit normal expansion and recruitment to the cornea during ocular HSV-1 infection. In support of our hypothesis that C3 and T cells jointly coordinate corneal sensation loss, frank corneal sensation loss was not observed following ocular HSV-1 infection in C3-sufficient, alpha-beta T cell receptor-deficient mice (TCRα-/-) which congenitally lack classical CD4+ and CD8+ T cells (Figure 2D). To investigate potential functional defects of C3-/- T cells in an in vivo context, CD3+ T cells were harvested from HSV-infected WT or C3-/- mice at day 8 p. i. and adoptively transferred into TCRα-/- mice. Six days following cell transfer, TCRα-/- recipients were infected with HSV-1 and corneal sensation monitored longitudinally. Adoptive transfer of CD3+ T cells from either WT or C3-/- donors evoked progressive corneal sensation loss in TCRα-/- recipients by day 8 p. i. (Figure 2D). These findings further corroborate that C3-/- T cells remain functional in vivo. Analysis of corneal sensation was not feasible beyond 8 days p. i. , as the TCRα-/- mice succumbed to herpetic encephalitis. However, engraftment of donor cells was confirmed by analysis of T cells in the eye-draining MLN of recipient mice by flow cytometry at day 8 p. i. The baseline lymphocyte counts in MLN from TCRα-/- controls likely reflect expansion of non-classical T cell populations (Viney et al. , 1994). An increase in total cell number was observed for CD4+ but not CD8+ T cells upon adoptive transfer of purified CD3+ T cells into TCRα-/- recipient mice (Figure 2—figure supplement 1A). Consistent with this observation, our data show that transfer of CD3+ T cells from HSV-infected mice have no appreciable impact on HSV-1 titers in the TG of TCRα-/- recipients by day 8 p. i. (Figure 2—figure supplement 1B). Notably, bulk transfer of HSV-specific CD8+ T cells has been shown to reduce viral burden in the peripheral nervous system of C57BL/6 mice during acute HSV-1 infection (Conrady et al. , 2009; Royer et al. , 2016). Our collective findings reveal that C3 and T cells are individually necessary and interdependent in the pathogenesis of HSV-1-associated corneal sensation loss. Whether by direct or indirect mechanisms, these data support a paradigm in which C3 activation and T cell engagement coordinate corneal nerve damage in herpetic keratitis. Our data show that T cells contribute to corneal sensation loss during acute HSV-1 infection, yet whether this pathology is dependent upon CD4+ or CD8+ T cells remained unclear. To better discern the contributions of each subset, CD4+ and CD8+ T cells were harvested from infected WT mice, transferred into separate groups of TCRα-/- mice, and recipient mice subsequently infected with HSV-1. Corneal sensation loss during acute HSV-1 infection was only observed upon reconstitution with CD4+ T cells (Figure 3A). Donor cell engraftment was confirmed by flow cytometry, although a statistically significant increase in cell number within the eye-draining MLN was only observed upon transfer of CD4+ T cells (Figure 3—figure supplement 1). The requirement for antigen specificity was subsequently evaluated during acute HSV-1 infection by monitoring corneal sensation in WT and OT-II transgenic mice that generate ovalbumin (OVA) -specific CD4 T cells. Transgenic OT-II mice did not exhibit corneal sensation loss following infection despite evidence of bystander CD4+ T cell activation and recruitment into the cornea (Figure 3B–D). Adoptive transfer of CD4+ T cells from HSV-infected WT and OT-II mice into TCRα-/- recipients corroborated these findings (Figure 3—figure supplement 2). Abrasion of the corneal epithelium, which is required to mediate infection in this model, induces local chemokine expression capable of recruiting T cells to the cornea even in the absence of viral infection (Liu et al. , 2012). Nonetheless, active corneal HSV-1 infection was necessary to provoke corneal sensation loss, as TCRα-/- mice reconstituted with CD4+ T cells harvested from HSV-infected WT mice did not elicit auto/allo-antigen-associated sensation loss within two weeks of corneal scratch injury (i. e. mock infection) (Figure 3—figure supplement 3). Collectively, our data show that complement C3 and antigen-specific CD4+ T cells are simultaneously necessary to drive corneal sensation loss during HSV-1 infection. These results strongly portend that a coordinated inflammatory axis exists involving C3 and antigen-specific CD4+ T cells, and that it is responsible for sensory neuropathy in herpetic keratitis. Complement-mediated tissue pathology can arise from complement activators synthesized by the liver (systemic complement) or in tissue microenvironments (local complement). While local complement activation and regulation have been reported in the cornea in health and disease (Bora et al. , 1993; Clark and Bishop, 2018; Mondino and Brady, 1981; Verhagen et al. , 1992), the expression profile and cellular sources of various complement components have not been investigated in the context of ocular HSV-1 infection. To this end, a modest array of complement component transcripts was evaluated by semiquantitative real-time PCR on corneal buttons from healthy and HSV-infected mice at day 2 and 7 p. i. Upregulation of complement effectors and anaphylatoxin receptors were noted including C3, C5, C3ar1, and C5ar1 (Figure 4A, B). Constitutive expression of complement receptor 2 (CD21) has been reported in the corneal epithelium (Levine et al. , 1990), but variances in its expression during HSV-1 infection were not statistically significant across the time points evaluated (Figure 4B). Likewise, no differences in the local expression of various complement regulators were observed in HSV-infected corneas aside from the C1-inhibitor SerpinG1 (Table 1, Figure 4C). Collectively, this expression profile favors local complement activation, as effectors are upregulated without proportional enhancement of pathway regulatory components. As C3 is the central component of the complement activation pathway, the cellular sources of complement C3 were evaluated in corneas infected with HSV-1. Monocytes and tissue macrophages are known sources of C3 (Einstein et al. , 1977; Lubbers et al. , 2017; Morgan and Gasque, 1997; Verschoor et al. , 2001), thus CSF1R+ cells from the peripheral blood of transgenic CSF1R-GFP mice (MAFIA) were utilized as the relative standard for C3 transcript expression. Expression of C3 was noted in CD45+ leukocytes isolated from infected corneas, yet detection of C3 expression was lost when CSF1R+ cells were removed from the total CD45+ pool. In addition, isolated EpCAM+ corneal epithelial cells expressed C3 at levels comparable to blood monocytes. However, the C3 expression level was greater in whole-cornea preparations than among individual cell fractions (Figure 4D). Taken together, our data show that both tissue-resident non-hematopoietic cells and resident/infiltrating CSF1R+ leukocytes contribute to local C3 expression in the cornea during acute HSV-1 infection. Ocular HSV-1 infection amplifies local complement gene expression, yet whether local control of complement activation can be harnessed to prevent corneal nerve damage has not been explored. From a clinical perspective, modulating complement activation at the ocular surface may be a viable therapeutic option. As a proof of concept, daily ocular cobra venom factor (CVF) treatment was explored as a putative method to deplete C3 and preserve corneal sensation during acute HSV-1 infection. Vehicle (PBS) -treated mice exhibited corneal sensation loss following HSV-1 infection, but CVF treatment preserved corneal sensation (Figure 5A). Protein levels of C3 remained near baseline in the cornea following CVF treatment during HSV-1 infection, yet vehicle-treated animals exhibited a 300–600% increase in C3 protein levels in the cornea at days 3 and 7 p. i. (Figure 5B). Ocular CVF treatment did not significantly impact systemic serum C3 concentrations throughout the study (Figure 5C). Ocular CVF treatment limited corneal edema during HSV-1 infection relative to the vehicle control (Figure 5D). Consistent with this observation, CVF-treated animals had less leukocytic infiltrate (CD45+) into the cornea at day 3 p. i. and, specifically, fewer infiltrating CSF1R+ cells (Figure 5E, F). By day 7 p. i. , no difference in total CD45+ or CSF1R+ cells were observed in the cornea, yet there was a reduction in the total number of infiltrating CD4+ T cells (Figure 5G). Despite the difference in CSF1R+ cell infiltration into the corneas at day 3 p. i. , HSV-1 titers were similar in the corneas and TG comparing vehicle- and CVF-treated animals (Figure 5—figure supplement 1A). By day 7 p. i. CVF-treated animals had less virus in the corneas and TG than vehicle controls (Figure 5—figure supplement 1B), suggesting that CVF may have unexpected antiviral effects. However, CVF treatment did not have any discernable impact on T cell expansion in the eye-draining MLN or the total number of circulating CXCR3+ CD4+ T cells in HSV-1 infected animals (Figure 5—figure supplement 2). Collectively, these findings imply that complement-targeted therapeutics could be tailored for ophthalmic use to limit tissue inflammation and preserve corneal sensation. While complement C3 and antigen-specific CD4+ T cells appear to coordinate corneal sensation loss in herpetic keratitis, it remained to be determined whether this phenomenon was specific to HSV-1 infection. To this end, corneal sensation was measured independent of infection in two T cell-dependent ocular surface inflammatory diseases. Murine models of allergic eye disease (AED) and ocular graft-versus-host disease (GVHD) were employed to further delineate the hypothesized role of T cells in coordinating corneal nerve damage (Herretes et al. , 2015; Lee et al. , 2015). First, AED was induced using an established systemic ovalbumin (OVA) -sensitization and ocular challenge model (Figure 6A) mimicking aspects of chronic keratoconjunctivitis (Ahadome et al. , 2016; Lee et al. , 2015). Clinical signs of ocular allergy developed as anticipated in OVA-challenged mice (Figure 6B), yet corneal sensation loss was not observed (Figure 6C). Consistent with development of AED, corneas from OVA-challenged mice exhibited CD3+ T cell infiltration by challenge day 7 (Figure 6D). However, frank corneal nerve loss was not observed in AED (Figure 6D). Morphometric analysis of confocal image stacks confirmed the preservation of corneal nerve density during AED (Figure 6E), although CD3+ T cell numbers were elevated in sensitized animals relative to healthy controls (Figure 6F). Flow cytometry was used to verify that the cornea-infiltrating T cells were predominately CD4+ in the AED model (Figure 6G). Although clinical data show that corneal nerve remodeling can occur in patients with chronic ocular allergy (Hu et al. , 2008; Le et al. , 2011), allergy symptoms were not accompanied by frank loss of corneal mechano-sensation in the AED animal model. Corneal sensation was also evaluated in a T cell-dependent allogeneic model of chronic GVHD with systemic and ocular manifestations following hematopoietic stem cell (HSC) transplantation (Perez et al. , 2016). This minor histocompatibility antigen mismatch model was established by transferring HSC-rich bone marrow (BM) and purified T cells from C57BL/6 (H2b) donors into lethally irradiated sex-matched C3. SW-H2b recipients. Recipient controls that receive BM without T cells recover and do not develop GVHD (Figure 7A). Onset of GVHD was established by transfer of BM with CD4+ T cells only, CD8+ T cells only, or both CD4+ and CD8+ T cells. Progressive corneal sensation loss was observed following transfer of CD4+ T cells with and without addition of CD8+ T cells. Addition of CD8+ T cells alone evoked a transient corneal sensation deficit that recovered by day 26 post-transplant. However, corneal sensation loss was not observed in the BM only control group (Figure 7B). External signs of ocular disease (see Table 2) were also apparent in groups receiving CD4+ T cells by the study endpoint (Figure 7C). The presence of tissue-infiltrating CD3+ T cells in corneas from animals with GVHD was verified by confocal microscopy at the study endpoint. Consistent with sensation loss, corneal nerve integrity was markedly reduced in groups receiving CD4+ T cells (Figure 7D). Morphometric analysis of confocal image stacks confirmed the CD4-dependent decrease in corneal nerve density (Figure 7E) and concomitant increase in total CD3+ cells (Figure 7F). Taken together, our data show for the first time that corneal sensation loss occurs early in ocular GVHD and that this pathology is instigated in part by allogeneic CD4+ T cells. The pharmacologic impact of local CVF treatment on ocular GVHD progression was consequently explored in order to identify whether the CD4+ T cell-associated corneal sensation loss observed in both herpetic keratitis and ocular GVHD shared a common C3-codependent pathomechanism. For these experiments, GVHD was established in C3. SW-H2b recipients by transfer of C57BL/6-derived BM and CD3+ T cells. Recipients from the BM only control and GVHD cohorts received ophthalmic treatment with either PBS or CVF (Figure 8A). Systemic disease progressed equally in both GVHD cohorts regardless of treatment (Figure 8B). Although CVF administration was limited to the eye, systemic serum C3 concentrations were lower in the GVHD cohort treated with CVF compared to the PBS-treated GVHD groups at the study endpoint. Nonetheless, the inverted CD4: CD8 T cell ratio (Herretes et al. , 2015) in the spleens and eye-draining mandibular lymph nodes of both groups of GVHD mice confirmed the presence of systemic disease (Figure 8D). Despite development of systemic disease, local CVF treatment preserved corneal nerve integrity and mechano-sensory function in ocular GVHD (Figure 8E, F). Morphometric analysis of confocal image stacks from the study endpoint corroborated the preservation of corneal nerve density in CVF-treated mice from the GVHD cohort (Figure 8G), although CVF did not reduce the number of total CD3+ cells during GVHD relative to PBS (Figure 8H). In addition, CVF treatment also limited GVHD-associated periocular disease in female but not in male mice (Figure 8—figure supplement 1). In summary, these preclinical models establish that CD4+ T cells and complement C3 coordinate corneal sensory nerve damage in both herpetic keratitis and ocular GHVD. Moreover, our data provide a proof of principle that complement-targeted therapeutics may limit the severity of immune-mediated sensory nerve damage at the ocular surface.
Mechanistic advances in our understanding of the complement cascade’s physiologic regulation and pathologic contributions in disease have predictably spawned substantial investments in complement-targeted drug development over the past decade (Harris et al. , 2018; Ricklin et al. , 2018; Tomlinson and Thurman, 2018). The ocular surface is a unique milieu in which pharmacologic modulation of the complement pathway may limit the severity of inflammatory disease and improve clinical outcomes. This study provides proof of concept that such interventions may have important clinical impacts on ocular surface disease, and specifically neuropathic sensory disorders affecting the cornea. Complement-targeted drug development for ophthalmic use has almost exclusively focused on AMD with some interest in neuromyelitis optica (NMO), Stargardt disease, and autoimmune uveitis (Harris et al. , 2018; Pittock et al. , 2013). By July 2019, there were no complement-specific therapeutics indicated for ocular surface use registered on www. clinicaltrials. gov (search strings: ‘complement’ / ‘C3’ / ‘C5’ / ‘eculizumab’ AND ‘cornea’, ‘conjunctiva’, ‘dry eye’, ‘keratitis’, OR ‘ocular surface’). Nonetheless, the complement pathway is implicated in the pathophysiology of several ocular surface diseases (Bora et al. , 2008). The current investigation provides an important advancement in this arena by demonstrating that dysregulated complement activation can specifically contribute to sensory nerve damage in the cornea. While corneal nerves are increasingly implicated in maintenance of corneal immune privilege (Paunicka et al. , 2015; Neelam et al. , 2018; Guzmán et al. , 2018), this study is the first to identify that C3 is involved in the pathobiology of corneal sensory nerve damage. However, the individual fates of damaged corneal nerves were not explored herein. Lessons from peripheral nerve injury in other settings indicate that some damaged nerves undergo apoptosis, yet effectively promoting functional regeneration of surviving neurons remains a major clinical hurtle (Doron-Mandel et al. , 2015; Menorca et al. , 2013; Scheib and Höke, 2013; Shacham-Silverberg et al. , 2018). Likewise, promoting functional regeneration of damaged corneal nerves is important for restoration of immune privilege and ocular surface health. Our approach evaluated sensory nerve damage in murine models of T cell-dependent ocular surface disease including HSV-1 keratitis, OVA-induced AED, and ocular GHVD. The lack of a sensory phenotype in the AED model was unanticipated in light of evidence that patients with severe allergy exhibit changes in corneal nerve morphology (Hu et al. , 2008; Le et al. , 2011; Leonardi et al. , 2012). This contrasting model underscores the context-dependency of sensation loss during corneal inflammation. While we focused on C3 in HSV-1 keratitis and ocular GVHD due to the direct link to overt corneal sensation loss, complement may still have an important role in mediating neurogenic hypersensitivities in AED. In addition to loss of mechano-sensory function, corneal nerve pathologies can involve a broad array of neuropathic clinical symptoms including dryness, itch, and pain (Andersen et al. , 2017). Inflammatory mediators facilitate neuropathic hypersensitivities, but the therapeutic potential of complement inhibition is often overlooked (Baral et al. , 2019; Fritzinger and Benjamin, 2016). Moreover, the cornea produces many neurotrophic factors that influence sensory innervation in health and disease (Sacchetti and Lambiase, 2017; Yu et al. , 2015). Given the involvement of C3 activation in sensory nerve damage, future investigation into crosstalk between complement and other neurotropic factors in the cornea is warranted. Furthermore, AED is driven primarily by a combined Th2/Th17 CD4 T cell response that differs from the Th1 bias observed in HSV-1 keratitis and ocular GVHD (Herretes et al. , 2015; Saban et al. , 2013; Tang and Hendricks, 1996). This suggests that Th1 cytokines such as IFNγ, IL-2, and lymphotoxin-alpha may be important in coordinating corneal sensation loss. Nonetheless, the unique inflammatory milieus and differential polarizations of cornea-infiltrating T cells across these disease models may also influence the downstream effects of complement activation in the tissue microenvironment (Tomlinson and Thurman, 2018). Future studies are needed to broaden the scope of these observations to other peripheral nerve diseases. For example, complement-mediated microvascular damage in diabetes correlates with neuropathy (Rasmussen et al. , 2018; Rosoklija et al. , 2000). While diabetic peripheral neuropathy can lead to neurotropic corneal ulcerations (Gao et al. , 2016b), the role of complement in diabetic corneal disease is currently unknown. Complement enhances clearance of infected cells and nascent virions during HSV-1 infection (Kostavasili et al. , 1997; Lubinski et al. , 2002; McNearney et al. , 1987). Importantly, HSV-1 can also evade C3 activation through endogenous expression of glycoprotein C (gC). By limiting C3b deposition on infected cells and nascent virions, gC can inhibit subsequent C5 binding and membrane attack complex (MAC) formation (Kostavasili et al. , 1997; Lubinski et al. , 2002; McNearney et al. , 1987; Tegla et al. , 2011). This could explain why herpetic keratitis is less severe in rabbits infected with a gC-deficient strain of HSV-1 during acute infection (Drolet et al. , 2004). However, this gC-mediated viral evasion strategy may also be cell type-dependent. Cell culture modeling indicates that neuronal cells maintain host-encoded complement regulator expression more efficiently and are more resistant to MAC deposition than epithelial cells upon HSV-1 infection (Rautemaa et al. , 2002). Accordingly, complement-mediated sensory nerve damage in HSV-1 keratitis may reflect a maladaptive outcome of host-defense. This is consistent with our data showing that immunologically naive C3-/- mice maintain corneal sensation and innervation, yet they exhibit concomitant enhancement of viral shedding in the tear film and increased viral burden in the TG by day 7 p. i. The absence of a concordant difference in HSV-1 titers in corneas from WT and C3-/- mice is likely explained by the potent antiviral effects of type-1 interferon (Conrady et al. , 2011; Royer and Carr, 2016). This suggests that the maintenance of corneal innervation in C3-/- mice enables enhanced shedding of nascent virions produced within the TG. Moreover, there is no difference in the amount of latent HSV-1 in the TG of naive WT and C3-/- mice 30 days after ocular HSV-1 challenge (Royer et al. , 2019). Taken together, this evidence indicates that although C3 is involved in progression of keratitis and sensory nerve fiber retraction during acute infection, it likely does not contribute to complete elimination of infected nerves. The studies reported herein involve immunologically naive mice, but the impacts of preexisting humoral immune responses on complement pathway activation and regulation in the cornea during HSV-1 infection remain incompletely understood. This point is of considerable importance clinically, as herpes-associated neurotropic keratitis typically develops as a result of recurrent corneal infections in patients (Hamrah et al. , 2010). Furthermore, we have recently reported that C3 is essential for optimal antibody-dependent viral clearance following ocular HSV-1 challenge in mice (Royer et al. , 2019; Royer et al. , 2017). While vaccinated animals did not exhibit corneal sensation loss following ocular HSV-1 infection in those studies, deposition of the terminal C3 cleavage product C3d was present in the corneal epithelium (Royer et al. , 2017). Host gene expression data herein corroborates that HSV-1 infection creates an imbalance in the local complement effector to regulator expression ratios that contribute to aberrant complement pathway activation in the cornea. This imbalance in ‘complement proteostasis’ may favor deposition of complement fragments and sublytic MAC on corneal sensory nerve fibers (Tegla et al. , 2011; Triantafilou et al. , 2013). Notwithstanding, the nerve-intrinsic molecular mechanisms responsible for trigeminal sensory fiber retraction, neuronal death, or axonal regeneration in the cornea are largely unknown (Stepp et al. , 2017). Future work is needed to identify the respective complement activation pathways involved in pathologic and protective immune responses to HSV-1 in the cornea. Identification of the complement pathway’s role in initiating corneal sensation loss following HSV-1 infection is an important advancement in the mechanistic understanding of this disease process, as it introduces an array of potential drug targets for local therapy. Previous observations note that complement activation in antigen-induced keratitis may be mediated by cellular immune mechanisms (Verhagen et al. , 1992). The identification of CSF1R+ macrophages/monocytes as a local source of C3 during infection (Figure 4D) is also critical, as these cells are recruited to the cornea in the early stages of HSV-1 infection and are associated with corneal nerve damage in HSV-1 keratitis (Chucair-Elliott et al. , 2017b; Conrady et al. , 2013). Moreover, CD4 T cells coordinate this pathology. Our data corroborate findings from the Hendricks’ lab showing that CD4 T cells are associated with corneal sensation loss in HSV-1 keratitis (Yun et al. , 2014), yet our adoptive transfer experiments establish that this process is not dependent upon endogenous C3 production by cornea-infiltrating donor T cells (Figure 2D). Notably, the tempo of HSV-associated sensation loss was consistent among models once initiated (Figure 1B, Figure 2D, Figure 3A). However, the initial onset was delayed by one day in the adoptive transfer model. This likely reflects the relative lymphopenic status of T cell-reconstituted TCRα-/- mice compared to WT mice (compare Figure 2B and Figure 3—figure supplement 1). Corneal sensation loss in HSV-1 infection and ocular GVHD shared a common mechanism dependent upon CD4+ T cells and complement C3. The role of intracellular C3 in regulating human Th1 responses has received much attention in recent years (Elvington et al. , 2017; Hansen et al. , 2019; Liszewski et al. , 2013). Such studies involve CD3 and CD46 stimulation to activate human CD4 T cells in vitro. However, mice do not express CD46. Instead, anaphylatoxin receptor signaling has been shown to modulate Th1 cytokine production in murine T cells (Strainic et al. , 2008). Our ex vivo re-stimulation data clearly show that there is no defect in IFNγ production by CD4 T cells from C3-/- mice following HSV-1 infection. Others have shown similar data based on T cell proliferation in response to re-stimulation with HSV-1 antigen (Da Costa et al. , 1999). In contrast, a reduction in IFNγ production by CD4 T cells from C3-/- mice was reported following in vitro expansion under Th1 polarizing conditions (Liszewski et al. , 2013). Nonetheless, it is possible that anaphylatoxin receptor signaling from locally produced C3a (and potentially C5a generated downstream) modulates T cell effector function upon extravasation into the inflamed cornea. The precise relationships between T cells and C3 in our mouse models of corneal neuropathic disease remain to be identified. Our working hypothesis involves an indirect mechanism whereby sublytic MAC deposition from local complement activation damages sensory nerves, which are then cleared by T cell-activated phagocytes or other cytolytic cells. These could include tissue-resident and infiltrating leukocytes (macrophages, monocytes, dendritic cells, and NK cells) as well as nerve-associated corneal epithelial cells (Buela and Hendricks, 2015; Chucair-Elliott et al. , 2017b; Gao et al. , 2016a; Koyama and Hill, 2016; Liu et al. , 2017; Royer et al. , 2018; Seyed-Razavi et al. , 2014; Stepp et al. , 2017). Emerging evidence indicates that the plasma membranes of corneal nerves and epithelial cells fuse thereby enabling cytosolic exchange (Stepp et al. , 2017). In light of this, it is plausible that complement-mediated damage to corneal epithelial cells has a direct impact on sensory nerves. Although corneal nerve damage is an established pathology in animal models of HSV-1 infection (Chucair-Elliott et al. , 2015; He et al. , 2017b; Yun et al. , 2014), this is the first report documenting loss of corneal mechano-sensory function in ocular GVHD. Notably, patients rarely exhibit neurological manifestations of GVHD affecting other tissues/organs (Grauer et al. , 2010). Corneal sensation loss may provide a clinical benchmark for initiation of targeted therapies to curb progression of ocular GVHD in patients. Data from our animal model of GVHD suggest that corneal sensation loss is an early warning sign of progressive ocular and systemic GVHD. Corneal sensation measurements are warranted in patients following HSCT to substantiate this finding among those who develop chronic GVHD. Published imaging studies confirm that corneal nerve remodeling occurs in patients with ocular GVHD (He et al. , 2017a; Tepelus et al. , 2017); therefore, clinical studies to delineate the kinetics of disease onset are needed. Complement C3 is a known driver of systemic GVHD (Kwan et al. , 2012; Ma et al. , 2014; Seignez et al. , 2017), and our data show that aberrant complement pathway activation also contributes to the pathogenesis of ocular GVHD including corneal sensation loss. Furthermore, localized CVF treatment preserved corneal sensation in both sexes during ocular GVHD, but treatment only abrogated other facets of ocular surface disease in female mice. This dimorphic outcome may reflect insufficient maintenance of C3 depletion in the ocular surface microenvironment, as animals were only treated twice weekly. Furthermore, complement activity is reportedly limited by terminal pathway components in female mice compared to males (Kotimaa et al. , 2016). Collectively, our data suggest that sensory nerve involvement may be a unique facet and treatment target in ocular GVHD. While the complement pathway has many components, C3 is the heart of the pathway and its activation products mediate virtually all downstream pathway functions. Accordingly, we used CVF to target complement activation in models of HSV-1 keratitis and ocular GVHD as a proof of concept for local delivery of complement-targeted therapeutics for ocular surface disease. In contrast to the neurotoxic effects of complete cobra venom, purified CVF is a ‘nontoxic’ derivative. CVF forms a biochemically stable convertase to rapidly hydrolyze mammalian C3 and C5. This ultimately results in complement inactivation via effector consumption (Vogel and Fritzinger, 2010). The only recorded side effect of purified CVF administration in rodents is transitory anaphylatoxin-mediated pulmonary inflammation resulting in acute respiratory distress (Proctor et al. , 2006; Vogel and Fritzinger, 2010). In our hands, respiratory distress was observed in some mice following the initial sub-conjunctival CVF injection irrespective of group assignment (animals were euthanized). However, topical CVF administration did not provoke respiratory or corneal abnormalities in any animals. Previous findings also show that topical CVF administration has no obvious effects on corneal health or transparency (Zaidi et al. , 2010). Experimental CVF administration was once thought to directly stimulate T cells, but such effects were later attributed to mitogen contamination (Rumjanek et al. , 1978; Cauvi et al. , 2012). Because our disease models were T cell-dependent, we utilized highly-purified CVF from a commercial source for our studies. Moreover, we found no evidence to suggest that CVF impacted T cell responses outside of the eye. Local CVF treatment reduced corneal T cell infiltration following HSV-1 infection (Figure 5G). However, this is likely due to reduced inflammation overall in C3-depleted corneas (Figure 5D). Likewise, ocular CVF treatment in the GVHD model did not impact on the total numbers of T cells in the corneas at the experiment endpoint. However, we have not excluded the possibility that CVF treatment may delay the tempo of T cell influx. Nonetheless, CVF administration preserved corneal sensation in both disease models. By addressing the ‘heart’ of the complement pathway (C3), this study opens the door to future investigation to further elucidate the relevant pathomechanisms and corresponding therapeutic targets. The relevant complement activation pathways (classical, alternative, lectin, terminal), cellular targets, and impacts of anaphylatoxin receptor signaling are topics of future investigation. Complement activation is a double-edged sword in the cornea, as activation can be either protective or harmful. Low-level complement turnover is observed in healthy corneas/tears, and complement may even contribute to the relative ‘paucibacterial’ state of the ocular surface microbiome (Doan et al. , 2016; McDermott, 2013; Willcox et al. , 1997). The mechanisms regulating each complement-mediated effect remain incompletely understood. Elucidation of how complement activation is triggered, the relevant molecular mechanisms of its downstream signaling, and contributions to pathology will promote an important advancement in drug development for ocular surface disease. Animal models are necessary for mechanistic studies when it comes to understanding complement pathway regulation, especially in dynamic tissue microenvironments; however, differences in complement regulation exist between mouse and man that will also have to be resolved to further assess the translational potential (Jacobson and Weis, 2008). This certainly applies to putative complement-targeted therapeutics for ocular surface treatment. Even among individual patients, small nucleotide polymorphisms evoke changes in complement activity that have major impacts on disease risks. Such genetic linkages, dubbed ‘complotype, ’ are important in differential susceptibility to AMD (Harris et al. , 2012; Paun et al. , 2016). Accordingly, complotype differences may help explain differential susceptibility patterns in a variety of ocular surface diseases. Our data herein have demonstrated successful prophylactic intervention in repressing complement-mediated sensory nerve pathology in two well characterized experimental T cell-dependent corneal disease models. Other avenues of future investigation are also needed to determine the efficacy of therapeutic intervention on established ocular surface diseases. Successful implementation of complement-targeted therapeutics for topical ophthalmic use may provide the benefit of controlling insidious ocular surface diseases without the risks associated with systemic therapies.
Mouse strains were originally purchased from The Jackson Laboratory (Bar Harbor, ME). These include: C57BL/6, C3-/- (stock #029661), TCRα-/- (stock # 002116), OT-II (stock #004194), MAFIA/CSF1R-GFP (stock #005070), C3. SW-H2b (stock #000438), and B6-GFP (stock #003291). Colonies were maintained in select-pathogen-free vivaria at the University of Oklahoma Health Sciences Center or Duke University Medical Center. Research was performed in accordance with protocols approved by respective institutional animal care and use committees. For all procedures relating to ocular HSV-1 infection, C57BL/6-background mice were anesthetized with ketamine and xylazine and euthanized by cardiac perfusion (Royer et al. , 2018). For infection, corneas were scratched to expose the epithelium and 1000 plaque forming units (PFU) of HSV-1 McKrae was applied to each eye. Viral stocks were propagated and infectious titers quantified by standard plaque assay on Vero cells (American Type Culture Collection, Manassas, VA) as previously reported (Royer et al. , 2015). For adoptive transfers, T cells were purified from splenocyte preparations using a BioRad S3e cell sorter (Hercules, CA). Corneal complement C3 depletion was achieved by subconjunctival injection of CVF (Cat. # 233552, Millipore Sigma, Burlington, MA) followed by topical maintenance dosing as described in respective figure legends. Allergic eye disease was initiated in B6 mice as previously described (Ahadome et al. , 2016; Lee et al. , 2015; Schlereth et al. , 2012). Briefly, hypersensitivity was elicited via intraperitoneal immunization with 10 μg ovalbumin (OVA) adjuvanted with 1 mg Alum and 300 ng pertussis toxin (all from Sigma-Aldrich Corp. , St. Louis, MO). Ocular allergy was subsequently induced via daily eyedrop challenge containing 250 μg OVA (Figure 6A). Clinical signs of AED were scored daily using established criteria (Schlereth et al. , 2012). Animals were euthanized by CO2 asphyxiation for tissue collection. Induction of GVHD was based on an established T-dependent, MHC-matched unrelated donor model (Perez et al. , 2016). Briefly, C3-SW. H2b mice were lethally irradiated (10. 5 Gy, Cs-137) and reconstituted with 5 × 106 T cell-depleted bone marrow (TCD-BM) cells from allogeneic C57BL/6 (H2b) mice. T cell depletion was achieved using BD anti-mouse CD90. 2 IMag particles (Cat. # 551518, San Jose, CA). Control groups received TCD-BM only, but GVHD onset required reconstitution with TCD-BM and T cells. T cells were isolated from B6 splenocytes using immunomagnetic microbeads (Miltenyi Biotec, San Diego, CA) targeting CD4 (Cat. #130-049-201), CD8 (Cat. #130-116-478), or CD90. 2 (Cat. #130-049-101) according to the manufacturer’s instructions. Cell purity was evaluated by flow cytometry (~80%) and adjusted such that 1. 3 × 106 CD4+ or CD8+ T cells or 2. 3 × 106 CD3+ T cells were injected into each recipient with TCD-BM. Irradiated animals received water containing gentamicin (6. 6 μg/ml) for ten days following irradiation (Henry Schein Animal Health, Dublin, OH). Supplical nutritional paste (Henry Schein) was also provided ad libitum to reduce weight loss. Systemic manifestations of GVHD were scored using established criteria (Perez et al. , 2016) and ocular disease scored according to Table 2. Corneal mechano-sensory function was measured on non-anesthetized mice with a Luneau Cochet-Bonnet esthesiometer (Western Ophthalmics, Lynwood, WA) in 0. 5 cm increments on a scale of 0 to 6 cm as previously described (Chucair-Elliott et al. , 2015). Sensation scores reflect the longest filament length capable of eliciting replicate blink reflexes when applied to the central cornea. A Bioptigen spectral domain optical coherence tomography (SD-OCT) system (Leica Microsystems, Buffalo Grove, IL) was utilized to evaluate corneal inflammation and edema in vivo (Downie et al. , 2014). Digital photography of the external eye was captured with a Leica MZ16 FA stereomicroscope. Corneal nerves were imaged in flat-mounted corneal buttons as described (Chucair-Elliott et al. , 2015). Briefly, corneas were harvested with the limbus intact, fixed, permeabilized, and labeled with Abcam anti-mouse beta-III tubulin primary antibody (Cat. # ab18207, Cambridge, MA) and a corresponding AlexaFluor647-conjugated secondary antibody (Cat. # 711-605-152, Jackson Immunoresearch, West Grove, PA). Corneas were also labeled with an eBioscience FITC-conjugated anti-mouse CD3ε antibody (ThermoFisher, Waltham, MA). Slides were imaged using an Olympus Fluoview1200 laser scanning confocal (Center Valley, PA) or a Nikon AR1 HD resonant scanning confocal (Melville, NY) microscope with sequential channel scanning. Confocal z-stack images were evaluated using Imaris software (Bitplane, Concord, MA) to quantify corneal nerves densities and the total numbers of CD3+ cells. Briefly, images were displayed as maximum intensity projections. Nerve volume per field of view was measured with the ‘create surface tool’ with manual thresholding for voxel area coverage of βIII tubulin+ fibers (default parameters; smooth surface area detail set to 0. 5 μm; background subtraction set to 8. 0 μm). Total CD3+ cells were quantified using the spots tool with default parameters, an estimated cell diameter of 8 μm, and classification using the quality filter method with manual thresholding. Morphometric nerve volume and cell count summary statistics were reported by the software per image (20x field of view). Nerve densities were normalized to the respective control group average. Secondary lymphoid organs were harvested and mechanically processed into single-cell suspensions (Royer et al. , 2015). Blood was collected from the facial vein and treated with erythrocyte lysis buffer prior to labeling. Corneas were digested in RPMI1640 media containing 0. 26 Wünsch units of Roche LiberaseTL enzyme blend (Sigma Aldrich) at 37° C as described (Royer et al. , 2017). Cell suspensions were filtered and labeled with Fc block and fluorochrome-conjugated antibodies (eBioscience). In vitro T cell functional assays were performed as previously described (Royer et al. , 2015). Briefly, cells were stimulated using 50 ng PMA and 800 ng ionomycin for three hours and monensin added after one hour (BD Biosciences). Intracellular IFNγ expression was evaluated by flow cytometry using a BD cytofix/cytoperm staining kit. Samples were analyzed on a Miltenyi Biotec MacsQuant10 or a BD LSRFortessa flow cytometer with MacsQuantify or FacsDiva software, respectively. For downstream gene expression studies, cornea digests were fractionated with Miltenyi microbeads by sequentially targeting CD45 (Cat. # 130-052-301) and EpCAM (Cat. # 130-105-958) as described (Royer et al. , 2018). Alternatively, GFP-expressing cells were purified or depleted using a BioRad S3e cell sorter. Tissues were harvested and homogenized in phosphate-buffered saline (PBS) containing 1x Calbiochem protease inhibitor cocktail (Millipore Sigma). Serum was obtained by collecting blood from the facial vein into BD microtainer serum separator tubes. Tissue homogenates and serum were clarified by centrifugation at 10,000×g. Chemokine concentrations were determined using a BioRad Luminex system and Millipore Milliplex MAP technology. Concentrations of C3 were measured by ELISA (Abcam, Cat. # ab157711). Gene expression studies were performed using RNA isolated from tissue and cells using the Trizol (ThermoFisher) method and converted to cDNA with iScript (BioRad). Real-time PCR was performed using PrimePCR technology with commercially validated primer sequences (Biorad) and a Biorad CFX-Connect thermocycler as directed. Relative expression was calculated using the 2–ΔΔCt method with GAPDH (glyceraldehyde 3-phosphate dehydrogenase) as a reference gene. Final PCR products were resolved on a 2% agarose gel and imaged with an Azure Biosystems C-series gel documentation system (Dublin, CA) to confirm C3 expression in cell subsets. All data were evaluated using Prism six software (GraphPad, San Diego, CA). Statistical tests and post hoc analyses utilized are listed in each figure legend. Significance thresholds are indicated as follows *=P < 0. 05, **=P < 0. 01, ***=P < 0. 001 for all data. Supporting data are available online. | Most people have likely experienced the discomfort of an eyelash falling onto the surface of their eye. Or that gritty sensation when dust blows into the eye and irritates the surface. These sensations are warnings from sensory nerves in the cornea, the transparent tissue that covers the iris and pupil. Corneal nerves help regulate blinking, and control production of the tear fluid that protects and lubricates the eye. But if the cornea suffers damage or infection, it can become inflamed. Long-lasting inflammation can damage the corneal nerves, leading to pain and vision loss. If scientists can identify how this happens, they may ultimately be able to prevent it. To this end, Royer et al. have used mice to study three causes of hard-to-treat corneal inflammation. The first is infection with herpes simplex virus (HSV-1), which also causes cold sores. The second is eye allergy, where the immune system overreacts to substances like pollen or pet dander. And the third is graft-versus-host disease (GVHD), an immune disorder that can affect people who receive a bone marrow transplant. Royer et al. showed that HSV-1 infection and GVHD – but not allergies – made the mouse cornea less sensitive to touch. Consistent with this, microscopy revealed damage to corneal nerves in the mice with HSV-1 infection and those with GVHD. Further experiments showed that immune cells called CD4 T cells and a protein called complement C3 were contributing to this nerve damage. Treating the mice with an experimental drug derived from cobra venom protected the cornea from the harmful effects of inflammation. It did so by blocking activation of complement C3 at the eye surface. Identifying factors such as complement C3 that are responsible for corneal nerve damage is an important first step in helping patients with inflammatory eye diseases. Many drugs that target the complement pathway are currently under development. Some of these drugs could potentially be adapted for delivery as eye drops. But first, experiments must test whether complement also contributes to corneal nerve damage in humans. If it does, work can then begin on testing these drugs for safety and efficacy in patients. | Abstract
Introduction
Results
Discussion
Materials and methods | microbiology and infectious disease
immunology and inflammation | 2019 | Complement and CD4+ T cells drive context-specific corneal sensory neuropathy | 14,453 | 490 |
Variation in the presentation of hereditary immunodeficiencies may be explained by genetic or environmental factors. Patients with mutations in HOIL1 (RBCK1) present with amylopectinosis-associated myopathy with or without hyper-inflammation and immunodeficiency. We report that barrier-raised HOIL-1-deficient mice exhibit amylopectin-like deposits in the myocardium but show minimal signs of hyper-inflammation. However, they show immunodeficiency upon acute infection with Listeria monocytogenes, Toxoplasma gondii or Citrobacter rodentium. Increased susceptibility to Listeria was due to HOIL-1 function in hematopoietic cells and macrophages in production of protective cytokines. In contrast, HOIL-1-deficient mice showed enhanced control of chronic Mycobacterium tuberculosis or murine γ-herpesvirus 68 (MHV68), and these infections conferred a hyper-inflammatory phenotype. Surprisingly, chronic infection with MHV68 complemented the immunodeficiency of HOIL-1, IL-6, Caspase-1 and Caspase-1; Caspase-11-deficient mice following Listeria infection. Thus chronic herpesvirus infection generates signs of auto-inflammation and complements genetic immunodeficiency in mutant mice, highlighting the importance of accounting for the virome in genotype-phenotype studies.
HOIL-1 (encoded by the RBCK1 gene), HOIP (RNF31) and SHARPIN form the linear ubiquitin chain assembly complex (LUBAC), which linearly ubiquitinates receptor signaling complex components such as NEMO to enhance NF-κB activation after engagement of immune receptors including TNF-R1, IL-1R, CD40, TLRs and NOD2 (Tokunaga et al. , 2011; Tokunaga et al. , 2009; Ikeda et al. , 2011; Gerlach et al. , 2011; Haas et al. , 2009; Zak et al. , 2011; Hostager et al. , 2011; Boisson et al. , 2012; Damgaard et al. , 2012; Tian et al. , 2007). Recently, HOIL-1/LUBAC was also shown to be important for activation of the NLRP3/ASC inflammasome in macrophages via linear ubiquitination of ASC (Rodgers et al. , 2014). These data suggest that HOIL-1 plays multiple roles in inflammation and infection. In mice, SHARPIN deficiency results in auto-inflammation involving multiple organs including the liver, esophagus, lung and, most noticeably, chronic proliferative dermatitis of the skin (Seymour et al. , 2007). The development and organization of secondary lymphoid organs and antibody isotype switching are also impaired in these mice (HogenEsch et al. , 1999). Loss of HOIP catalytic activity in B cells results in the impaired development of B1 B cells and antibody responses to antigen (Sasaki et al. , 2013). However, HOIL-1-deficient mice have not been analyzed extensively to date. Sixteen patients with bi-allelic mutations in the gene encoding HOIL-1 have been reported (Boisson et al. , 2012; Nilsson et al. , 2013; Wang et al. , 2013). Three patients exhibited cardiomyopathy, amylopectinosis, hyper-inflammation and mild immunodeficiency associated with an increased frequency of bacterial infections, whereas other patients presented with amylopectinosis and myopathy alone (Figure 1—figure supplement 1). The role of HOIL-1 in inflammation and immunity to infection in vivo is, therefore, uncertain. Although there are multiple possible explanations for the variable clinical presentations of the reported patients including hypomorphic expression of HOIL-1 or effects of mutations on protein function, another possibility was that environmental factors alter the clinical presentation of HOIL-1 deficiency. In this study we define the function of HOIL-1 in murine immunity to infection and explore the potential role of the virome in determining HOIL-1 deficiency-associated phenotypes. The bacterial microbiome and the virome regulate inflammation and immunity (Virgin, 2014; Virgin et al. , 2009; Belkaid and Hand, 2014). Within the virome, herpesviruses persistently infect most humans, and exert significant effects on innate immunity in mice during experimental chronic infection, including increasing resistance to tumors and a range of pathogens (Barton et al. , 2007; White et al. , 2010; Yager et al. , 2009; Nguyen et al. , 2008; Haque et al. , 2004). However, the potential effects of chronic infection on the phenotypic manifestations of immune deficiencies have not been considered. In this study, we show that chronic herpesvirus infection can alter the presentation of several genetic immunodeficiencies in mice. We first found that, in naïve mice, HOIL-1 is essential during infection with Listeria monocytogenes, Toxoplasma gondii and Citrobacter rodentium and for efficient induction of pro-inflammatory cytokines that are known to be essential for resistance to lethal infection by hematopoietic cells during Listeria infection. In contrast, HOIL-1 knock-out (KO) mice, with null mutations in the Rbck1 gene that encodes HOIL-1, were resistant to infection with murine γ-herpesvirus 68 (MHV68) and Mycobacterium tuberculosis. Although HOIL-1 KO mice raised in a barrier facility did not display signs of auto-inflammation, chronic infection with MHV68 or M. tuberculosis resulted in elevated inflammatory cytokines circulating in the serum, similar to that observed in some patients with mutations in RBCK1 (HOIL1). Interestingly, latent infection with MHV68 rescued HOIL-1 deficient mice from lethality during Listeria infection and induced high levels of the protective cytokine, interferon-gamma (IFNγ). MHV68 latency also protected IL-6, Caspase-1 and Caspase-1; Caspase-11 deficient mice from Listeria-induced lethality, indicating that the ability of latent infection to complement a genetic immunodeficiency is not restricted to mutation of Hoil-1. These data indicate that chronic infections can modify the clinical presentations of genetic variations, thereby opening a new avenue for the analysis and interpretation of human genotype-phenotype association studies. We speculate that the protective effect of chronic herpesvirus infection is due to the stimulation of the function of the innate immune system in a manner that compensates for deficient early cytokine responses associated with multiple immunodeficiencies.
HOIL-1 KO mice (Tokunaga et al. , 2009) were born at Mendelian ratios and, in contrast to SHARPIN-deficient mice, failed to develop TNFα-driven inflammatory skin disease (Ikeda et al. , 2011; Gerlach et al. , 2011; Tokunaga et al. , 2011; Tokunaga and Iwai, 2012) and exhibited normal histology of lymphoid organs, liver, lung, and kidney, and the presence of Peyer' s patches along the small intestine (not shown). Aged HOIL-1 KO mice exhibited deposits of material that stained with periodic acid-Schiff reagent and was resistant to digestion with diastase, similar to the amylopectin-like material observed in humans with HOIL-1 deficiency (Figure 1—figure supplement 2) (Boisson et al. , 2012). Importantly, these barrier-raised mice showed minimal signs of baseline hyper-inflammation. In this regard, HOIL-1 KO mice exhibited normal numbers of lymphoid and myeloid cells in the spleen and thymus, normal complete blood counts (Figure 1—figure supplement 3A, B, D), and no detectable increase of tumor necrosis factor alpha (TNFα) or interleukin 6 (IL-6) in serum (discussed below). However, in the peritoneum, HOIL-1 KO mice contained about twofold more B cells, T cells and resident macrophages without changes in other cell types (Figure 1—figure supplement 3C). Expression of neighboring genes, Trib3 and Tbc1d20, was unaffected by disruption of the Rbck1 (Hoil1) gene (Figure 1—figure supplement 4). To determine the requirement for HOIL-1 during the immune response to infection in vivo, we challenged HOIL-1 KO mice with a number of different pathogens. Strikingly, HOIL-1 KO mice were highly susceptible to even low dose infection with the facultative gram-positive intracellular bacterium, Listeria monocytogenes (Listeria), with 80%, 80% and 50% of mice succumbing to infection within 10 days of intraperitoneal (i. p.) inoculation with 105,104 and 103 CFU, respectively (Figure 1A). Although bacterial burdens in the spleens and livers of control and HOIL-1 KO mice were similar 1 and 3 days post-infection with 105 CFU, bacterial CFUs were elevated in HOIL-1 KO mice by 6 days post-infection, indicating that HOIL-1 KO mice were unable to control and clear the bacteria (Figure 1B). Further, these mutant mice developed large inflammatory lesions in the liver, elevated liver enzymes in the serum, and widespread tissue destruction in the spleen (Figure 1—figure supplement 5, not shown). 10. 7554/eLife. 04494. 006Figure 1. HOIL-1 KO mice are highly susceptible to acute infection with Listeria monocytogenes, Toxoplasma gondii and Citrobacter rodentium. (A) Survival of control (blue circles) and HOIL-1 KO (red squares) mice following i. p. inoculation with 105 (left panel; control n = 35, HOIL-1 KO n = 19), 104 (middle panel; control n = 15, HOIL-1 KO n = 15) or 103 (right panel; control n = 15, HOIL-1 KO n = 15) CFU Listeria strain EGD. (B) Listeria CFU in spleen and liver from control (blue circles) and HOIL-1 KO (red squares) mice infected with 105 CFU i. p. for 1 day (left panel), 3 days (middle panel) or 6 days (right panel). Each symbol represents an individual mouse and the mean log10 CFU is indicated. The dashed line indicates the limit of detection. (C) Survival of control (blue circles) and HOIL-1 KO (red squares) mice following inoculation with 5000 (left panel; control n = 17, HOIL-1 KO n = 5) or 100 (middle panel; control n = 10, HOIL-1 KO n = 10) tachyzoites T. gondii strain Pru-luc. (D) Log10 total flux (luciferase activity; photons per second) as a measure of parasite burden 8 days post-infection with 100 tachyzoites. Each symbol represents an individual mouse and the mean log10 is indicated. (E, F) Survival (E) and weight (F) of control (blue circles) and HOIL-1 KO (red squares) mice following oral gavage with 2 × 109 CFU C. rodentium. n = 20/group for survival and n = 10/group for weight. *p ≤ 0. 05, **p ≤ 0. 01, ***p ≤ 0. 001, ****p ≤ 0. 0001. Statistical analyses were performed using logrank Mantel–Cox test (A, C and E), Mann–Whitney test (B), or t-test (D). DOI: http: //dx. doi. org/10. 7554/eLife. 04494. 00610. 7554/eLife. 04494. 007Figure 1—figure supplement 1. Comparison of RBCK1/HOIL1 alleles from RBCK1/HOIL1-mutant patients. Npl4 zinc finger ubiquitin binding domain, RING; really interesting new gene E3 ligase domain, IBR; Inbetween-RING domain. HOIL1L/RBCK1 isoform 2 (reference sequences NM_031229. 2/NP_112506. 2) was used for annotation. DOI: http: //dx. doi. org/10. 7554/eLife. 04494. 00710. 7554/eLife. 04494. 008Figure 1—figure supplement 2. Myocardium from aged HOIL-1 KO mice contains amylopectin-like deposits. Representative PAS (top), PAS plus diastase digestion (middle) and H&E-stained sections of myocardium from 18 month-old HOIL-1 KO (right) and control (left) mice. The scale bar (inset) represents 100 µm. DOI: http: //dx. doi. org/10. 7554/eLife. 04494. 00810. 7554/eLife. 04494. 009Figure 1—figure supplement 3. Analysis of hematopoietic cell populations from naïve HOIL-1 KO mice. (A–C) Flow cytometric analysis of cell populations in the spleen (A), thymus (B) and peritoneum (C) of HOIL-1 KO (red squares) and control (blue circles) mice. DP; CD4, CD8 double-positive. (D) Complete blood counts from HOIL-1 KO (red squares) and control (blue circles) mice. WBC, white blood cells, ×103/mm3, RBC, red blood cells, ×106/mm3; HGB, hemoglobin, g/dl; HCT, hematocrit, %; MCV, mean corpuscular volume, µm3; MCH, mean corpuscular hemoglobin, pg; MCHC, mean corpuscular hemoglobin concentration, %; Seg Neu, segmented neutrophils, %; Lymphos, lymphocytes, %, Monos, monocytes, %. Eosinophils, basophils or band neutrophils were not detected. Each symbol represents an individual mouse and the mean is indicated. DOI: http: //dx. doi. org/10. 7554/eLife. 04494. 00910. 7554/eLife. 04494. 010Figure 1—figure supplement 4. Hoil1/Rbck1 and neighboring gene (Trib3 and Tbc1d20) transcript expression in control and HOIL-1 KO bone marrow derived macrophages. Data represent the mean ± SEM from cells derived from four pairs of mice each analyzed in triplicate. *p ≤ 0. 05, **p ≤ 0. 01, ***p ≤ 0. 001, ****p ≤ 0. 0001. Statistical analyses were performed using t-test. DOI: http: //dx. doi. org/10. 7554/eLife. 04494. 01010. 7554/eLife. 04494. 011Figure 1—figure supplement 5. Pathology of HOIL-1 KO mice during Listeria infection. Alanine aminotransferase (ATL; left panel) and aspartate aminotransferase (AST; right panel) levels in serum from control (blue circles) and HOIL-1 KO (red squares) mice 6 days after infection with 105 CFU Listeria. Each symbol represents an individual mouse and the mean is indicated. *p ≤ 0. 05, **p ≤ 0. 01; t-test with Welch' s correction. DOI: http: //dx. doi. org/10. 7554/eLife. 04494. 011 HOIL-1 KO mice were also highly susceptible to infection with a relatively avirulent type II strain of the intracellular apicomplexan parasite Toxoplasma gondii (T. gondii) (Figure 1C). Despite infection with 5000 parasites resulting in lethality in only 20% of control mice, 100 parasites was sufficient to induce lethality in 100% of HOIL-1 KO mice. Quantification of parasite-encoded luciferase expression in vivo revealed that HOIL-1 KO mice failed to control T. gondii replication by 8 days post-infection (Figure 1D). HOIL-1 KO mice also succumbed to infection with the enteric gram-negative pathogen Citrobacter rodentium, whereas control mice were highly resistant (Figure 1E, F). These data indicated that loss of HOIL-1 expression confers profound immunodeficiency in barrier-raised mice. To define the role of HOIL-1 in immunity, we examined the response to Listeria in more detail. One patient with HOIL-1-associated immunodeficiency showed signs of recovery from hyper-inflammation after hematopoietic stem cell transplantation (Boisson et al. , 2012). In mice, reciprocal bone marrow transplantation revealed that expression of HOIL-1 in radiation-sensitive hematopoietic cells was critical for resistance to Listeria (Figure 2A, Figure 2—figure supplement 1). We noted that control mice that received control bone marrow were slightly more susceptible to infection than non-irradiated control mice (compare with Figure 1A), suggesting that reconstitution does not fully restore the immune system of a lethally irradiated mouse to that of a non-irradiated animal. Despite this caveat, irradiated wild-type control mice that received HOIL-1 KO bone marrow and were challenged with 105 Listeria 8 weeks later succumbed to infection at the same rate as HOIL-1 KO mice that had received HOIL-1 KO bone marrow. HOIL-1 KO mice that received control bone marrow had an increased survival rate, but still succumbed more readily than control mice that received control bone marrow. These data indicate that, while HOIL-1 expression is essential in bone marrow-derived cells, HOIL-1 may also play a role in radiation resistant cells during Listeria infection. 10. 7554/eLife. 04494. 012Figure 2. HOIL-1 is required in an innate immune cell compartment during Listeria infection. (A) Survival of control and HOIL-1 KO reciprocal bone marrow chimeric mice following infection with 105 CFU Listeria. *p ≤ 0. 0083; logrank Mantel–Cox test corrected for multiple comparisons. (B) Survival of RAG1 KO HOIL-1 WT (blue circles; n = 12) and RAG1 KO HOIL-1 KO (red squares; n=11) mice following infection with 104 CFU Listeria. (C) Listeria CFU in spleen and liver from RAG1 KO HOIL-1 WT (blue circles) and RAG1 KO HOIL-1 KO (red squares) mice infected with 104 CFU for 3 days. Each symbol represents an individual mouse and the mean log10 is indicated. For B and C, *p ≤ 0. 05, **p ≤ 0. 01, ***p ≤ 0. 001, ****p ≤ 0. 0001; logrank Mantel–Cox test and Mann–Whitney test, respectively. DOI: http: //dx. doi. org/10. 7554/eLife. 04494. 01210. 7554/eLife. 04494. 013Figure 2—figure supplement 1. Confirmation of hematopoietic reconstitution of bone marrow chimeric mice. Percent Hoil1/Rbck1+/+ (Hoil1/Rbck1 intron 7; top panel) and percent Hoil1/Rbck1−/− (neomycin-resistance cassette; bottom panel) genomic DNA (gDNA) in peripheral blood from control and HOIL-1 KO reciprocal bone marrow chimeric mice determined by qPCR. DOI: http: //dx. doi. org/10. 7554/eLife. 04494. 01310. 7554/eLife. 04494. 014Figure 2—figure supplement 2. HOIL-1 KO mice are capable of generating an adaptive immune response to Listeria. Listeria titers in spleen and liver of naïve (circles) or pre-immunized (103 CFU for 28 days, squares) control (blue symbols) and HOIL-1 KO (red symbols) mice challenged with 106 CFU Listeria for 3 days. *p ≤ 0. 05, **p ≤ 0. 01, ***p ≤ 0. 001, ****p ≤ 0. 0001. Statistical analyses were performed using two-way ANOVA with Holm-Sidak' s multiple comparison test. DOI: http: //dx. doi. org/10. 7554/eLife. 04494. 014 To determine whether HOIL-1 deficiency resulted in a defect in innate or adaptive immunity, we bred the HOIL-1 KO mice onto a RAG1-deficient background. T and B cell-deficient RAG1 HOIL-1 double KO mice succumbed to infection significantly faster than RAG1 KO mice (Figure 2B), and exhibited elevated bacterial burden in the spleen and liver 3 days post-infection (Figure 2C), indicating that HOIL-1 plays an essential role in innate immunity during Listeria infection. Indeed, HOIL-1-deficient mice succumbed to infection at the same rate regardless of the presence or absence of the adaptive immune system (compare Figures 1A and 2B). Further, HOIL-1 KO mice immunized with a low dose of Listeria were capable of mounting a protective adaptive response to a high dose secondary challenge with Listeria 28 days later (Figure 2—figure supplement 2). We noted that 1000 CFU administered i. p. was a borderline dose required to successfully immunize control mice in our experiments, despite being sufficient to induce lethality in 50% of HOIL-1 KO mice (Figure 1A). Together, these data do not rule out a role for HOIL-1 in adaptive immunity, but show that HOIL-1 plays a major role in hematopoietic cells to foster innate immunity to Listeria infection. Innate immunity to Listeria in mice depends on tissue-resident macrophages and CD8α+ dendritic cells responding to Listeria infection by secreting pro-inflammatory cytokines including TNFα, IL-12, and IL-6. These cytokines are each well recognized to be essential for survival after Listeria infection (Unanue, 1997; Williams et al. , 2012) through their role in coordinating activation of NK cells, NKT cell and T cells to produce IFNγ required for the bactericidal activity of phagocytic cells. Therefore, to further define a role for HOIL-1 in the innate immune system, we determined whether HOIL-1 KO bone marrow-derived macrophages (macrophages herein) produced cytokines in response to Listeria infection with or without IFNγ treatment. Compared to control cells, HOIL-1 KO macrophages secreted only 50%, 20% and 10% of the expected levels of TNFα, IL-6 and IL-12p70 protein, respectively (Figure 3A). Consistent with a role for HOIL-1 in the activation of the NF-κB transcription factor following TLR stimulation, Listeria-infected HOIL-1 KO macrophages expressed decreased levels of Tnf, Il6 and Il12b mRNA (Figure 3B). The defects in cytokine transcript levels were of smaller magnitude than the decreases in secreted protein, particularly for Tnf, suggesting that HOIL-1 may also be involved in cytokine translation or secretion. However, HOIL-1 KO macrophages killed Listeria after activation with IFNγ as effectively as control cells, indicating the selectivity of HOIL-1 effects on macrophage function (Figure 3—figure supplement 1). 10. 7554/eLife. 04494. 015Figure 3. HOIL-1 is important for induction of pro-inflammatory cytokines following Listeria infection. (A) TNFα (6 hr), IL-6 (6 hr) and IL-12p70 (24 hr) protein in macrophage culture supernatants following infection with Listeria (LM) ± IFNγ co-treatment. (B) Induction of Tnf, Il6 and Il12b transcripts in macrophages over 12 hr following infection with Listeria plus IFNγ. Data represent the mean ± SEM of macrophages derived from two mice per genotype analyzed in triplicate and are representative of at least three independent experiments. (C) Induction of cytokine transcripts in peritoneal cells over 12 hr following infection of control (blue circles) and HOIL-1 KO (red squares) mice with 105 Listeria. Each symbol represents an individual mouse. *p ≤ 0. 05, **p ≤ 0. 01, ***p ≤ 0. 001, ****p ≤ 0. 0001. Statistical analyses were performed using t-test. DOI: http: //dx. doi. org/10. 7554/eLife. 04494. 01510. 7554/eLife. 04494. 016Figure 3—figure supplement 1. HOIL-1 is not required for listericidal activity of bone marrow-derived macrophages. Growth and killing of Listeria in untreated or IFNγ-pre-treated control and HOIL-1 KO macrophages at 0 and 6 hr post-infection. Data are from two independent experiments performed in duplicate. DOI: http: //dx. doi. org/10. 7554/eLife. 04494. 01610. 7554/eLife. 04494. 017Figure 3—figure supplement 2. Analysis of peritoneal cell populations following Listeria infection. Flow cytometric analysis of peritoneal cell populations in control (blue circles; 0 hr n = 20,6 hr n = 10,12 hr n = 7) and HOIL-1 KO mice (red squares; 0 hr n = 9,6 hr n = 12,12 hr n = 7) over 12 hr after infection with 105 CFU i. p. Data represent the mean ± SEM. *p ≤ 0. 05, **p ≤ 0. 01, ***p ≤ 0. 001, ****p ≤ 0. 0001. Statistical analyses were performed using t-test. DOI: http: //dx. doi. org/10. 7554/eLife. 04494. 01710. 7554/eLife. 04494. 018Figure 3—figure supplement 3. HOIL-1 is important for induction of pro-inflammatory cytokines by innate cells following Listeria infection in vivo. Induction of cytokine transcripts in peritoneal cells from uninfected (0 hr) RAG1 KO HOIL-1 WT (blue circles) and RAG1 KO HOIL-1 KO (red squares) mice or 3 hr after infection with 104 Listeria. Each symbol represents an individual mouse. DOI: http: //dx. doi. org/10. 7554/eLife. 04494. 018 We confirmed that induction of Tnf and Il6 mRNA was significantly impaired following Listeria infection in vivo by measuring cytokine transcripts in peritoneal cells from mice 3 to 12 hr after infection (Figure 3C). This reduction in cytokine transcripts could not be explained by a decrease in macrophage numbers (Figure 3—figure supplement 2). Surprisingly, Il12b transcript levels were similar in HOIL-1 KO mice, with a significant difference being detected at only 6 hr after infection. Similar decreases in Tnf and Il6 mRNA were observed in mice on the Rag1−/− background at 3 hr post-infection, confirming that these differences are due to a defect in innate immunity in the absence of HOIL-1 (Figure 3—figure supplement 3). We also noted that fewer NK cells and neutrophils were present in the peritoneum 6 hr after Listeria infection, suggesting delayed recruitment or proliferation of these cell types (Figure 3—figure supplement 2). These decreases in cytokine production and delayed cell recruitment likely synergize with defects in IL-1β and TNFα signaling observed by others (Tian et al. , 2007; Haas et al. , 2009; Tokunaga et al. , 2009,2011) to compromise antibacterial immunity, and may contribute to the impaired induction of Ifng mRNA observed by 12 hr (Figure 3C). These data indicate that HOIL-1 plays a critical role in coordinating essential early cytokine responses after Listeria infection. The above data demonstrate that HOIL-1 KO mice have a severe immunodeficiency after certain types of infection. To assess the generality of this phenotype we infected HOIL-1 KO mice with murine γ-herpesvirus 68 (MHV68), a genetic relative of the common persistent human herpesviruses, Epstein–Barr virus and Kaposi' s sarcoma-associated herpesvirus (EBV, KSHV) (Barton et al. , 2011; Speck and Ganem, 2010). HOIL-1 KO mice survived MHV68 infection for at least 3 months. MHV68 replication was unaffected by HOIL-1 deficiency in cultured macrophages, and was suppressed only slightly in vivo (Figure 4—figure supplement 1). Despite normal establishment of latency as determined by the number of cells carrying MHV68 genome 28 days after infection (Figure 4—figure supplement 2), the efficiency of MHV68 reactivation from latency in explanted peritoneal cells was significantly impaired (approximately 50-fold, Figure 4A). Similarly, HOIL-1 KO mice failed to succumb to infection with M. tuberculosis over 70 days of infection, and in fact exhibited lower bacterial colony counts in the spleen while counts in the lung were no different than controls (Figure 4B). Thus HOIL-1 KO mice are fully able to control, and may have an enhanced ability to control, specific aspects of acute and chronic MHV68 and M. tuberculosis infection, in striking contrast to the immunodeficiency apparent after infection with Listeria, Toxoplasma, and Citrobacter. 10. 7554/eLife. 04494. 003Figure 4. Enhanced inflammatory response and control of MHV68 and M. tuberculosis by HOIL-1 KO mice. (A) Limiting dilution assay of peritoneal cells from control (blue circles) and HOIL-1 KO (red squares) mice infected with MHV68 for 28 days onto mouse embryonic fibroblast monolayers to measure the frequency of cells capable of MHV68 reactivation. The dashed line indicates 63. 2%, which was used to determine the frequency of cells reactivating virus by the Poisson distribution. Data represent the mean from three independent experiments each with cells combined from three mice/group. *p ≤ 0. 05. Statistical analyses were performed by calculating the number of control and HOIL-1 KO cells required for 63. 2% of wells to contain complete cytopathic effect for each individual experiment by non-linear regression, then comparing these values by paired t-test. Preformed virus was not detected in disrupted samples (not shown). (B) M. tuberculosis titers in the lung and spleen of HOIL-1 KO (red squares) and control (blue circles) mice 70 days post-infection. *p ≤ 0. 05. Statistical analyses were performed using t-test. (C) TNFα, IL-6, IL-12/IL-23p40 and IFNγ protein detected in serum from naïve or latently-infected (28 days) control (blue circles) and HOIL-1 KO (red squares) mice. Each symbol represents an individual mouse and the mean is indicated. *p ≤ 0. 05, t-test with Welch' s correction (IL-12/IL-23p40) or Mann Whitney test (TNFα, IL-6, IFNγ). (D) TNFα, IL-6, IL-12/IL-23p40 and IFNγ protein in serum from mice from (B). Each symbol represents an individual mouse. Data are combined from two independent experiments. *p ≤ 0. 05, **p ≤ 0. 01. Statistical analyses were performed using t-test (TNFα, IL-12p40) with Welch' s correction (IFNγ) or Mann Whitney test (IL-6). DOI: http: //dx. doi. org/10. 7554/eLife. 04494. 00310. 7554/eLife. 04494. 004Figure 4—figure supplement 1. Acute MHV68 replication in vitro and in vivo is minimally affected by HOIL-1-deficiency. (A) MHV68 growth in HOIL-1 KO (red symbols) and control (blue symbols) bone marrow-derived macrophages with (squares) or without (circles) IFNγ pre- and post-treatment. Data represent the mean ± SEM from three independent experiments performed in triplicate. (B) MHV68 titers in spleen during acute infection of HOIL-1 KO (red squares) and control (blue circles) mice. **p ≤ 0. 01, Mann Whitney test. The dashed line indicates the limit of detection. DOI: http: //dx. doi. org/10. 7554/eLife. 04494. 00410. 7554/eLife. 04494. 005Figure 4—figure supplement 2. Establishment of MHV68 latency is similar in control and HOIL-1 KO mice. Limiting dilution PCR to determine the frequency of peritoneal cells from latently infected (28 days) HOIL-1 KO (red squares) and control (blue circles) mice containing MHV68 genomes. The dashed line indicates 63. 2%, which was used to determine the frequency of cells containing viral genome by the Poisson distribution. Data represent the mean from three independent experiments each with cells combined from three mice/group. Statistical analyses were performed by calculating the number of control and HOIL-1 KO cells required for 63. 2% of reactions to be positive for viral genome for each individual experiment by non-linear regression, then comparing these values by paired t-test. DOI: http: //dx. doi. org/10. 7554/eLife. 04494. 005 The ability of HOIL-1 KO mice to effectively control chronic herpesvirus infection allowed us to test the hypothesis that persistent virus infection might alter two phenotypes, hyper-inflammation and immunodeficiency, in which HOIL-1 KO mice appear to differ from some reported patients with bi-allelic mutations in RBCK1 (HOIL1) (Boisson et al. , 2012). Notably, patients with HOIL-1 deficiency and hyper-inflammation exhibited increased expression of IL-6 and TNFα in the serum and increased expression of mRNA for Il6 in blood cells (Boisson et al. , 2012). We therefore examined the serum of MHV68-infected HOIL-1 KO mice for cytokines essential for resistance to Listeria but deficient in Listeria-infected HOIL-1 KO mice. As previously observed (Barton et al. , 2007), latent infection of control mice with MHV68 was associated with an increase in circulating levels of TNFα, IL-6, IL-12 and IFNγ compared with uninfected mice (Figure 4C). In HOIL-1 KO mice, MHV68 latently resulted in small but significant increases in TNFα, IL-6 and IL-12p70 levels, and a more striking increase in IFNγ levels compared to latently infected controls. HOIL-1 KO mice chronically infected with M. tuberculosis also exhibited increased expression of both IL-6 and TNFα in serum at 70 days post-infection (Figure 4D). Therefore, there is an overlap between cytokines expressed in hyper-inflammatory patients and in chronically-infected HOIL-1 KO mice. MHV68 latency has been shown previously to induce symbiotic protection against Listeria infection in wild-type mice (Barton et al. , 2007). Because expression of TNFα, IL-6 and IFNγ are essential for control of Listeria infection in mice (Kopf et al. , 1994; Unanue, 1997; Williams et al. , 2012), and were impaired in Listeria-infected HOIL-1 KO mice (Figure 3C), but elevated in MHV68-infected HOIL-1 KO mice, we considered whether chronic MHV68 infection could complement the profound immunodeficiency observed in Listeria-infected barrier-raised HOIL-1 KO mice. As observed previously (Barton et al. , 2007), MHV68 latency, 1 month after infection, protected control mice from an otherwise lethal dose of Listeria (Figure 5A). MHV68 latency also protected HOIL-1 KO mice from a dose of Listeria at least 1000-fold higher than the LD50 for MHV68-negative mice (Figures 5A and 1A). Both control and HOIL-1 KO mice were still partially protected from Listeria challenge 6 months after MHV68 infection (Figure 5—figure supplement 1). A viral mutant capable of acute lytic infection but unable to efficiently establish latency (ORF73. stop) (Moorman et al. , 2003) was unable to efficiently protect HOIL-1 KO or control mice from Listeria infection, demonstrating that latent MHV68 is required to complement HOIL-1-associated immunodeficiency to Listeria (Figure 5B). 10. 7554/eLife. 04494. 019Figure 5. MHV68 latency rescues HOIL-1 KO, IL-6, Caspase-1 and Caspase-1; Caspase-11-deficient mice from Listeria-induced lethality. (A) Survival of control (blue symbols; mock n = 9, MHV68 n = 15) and HOIL-1 KO (red symbols; mock n = 10, MHV68 n = 20) mice challenged with 106 CFU Listeria 28 days following mock infection (circles) or infection with 106 PFU MHV68 (squares). *p ≤ 0. 0083; logrank Mantel–Cox test corrected for multiple comparisons. (B) Survival of control (blue symbols) and HOIL-1 KO (red symbols) mice challenged with 106 CFU Listeria 28 days following intranasal mock infection (circles) or infection with 5 × 104 PFU wild-type (squares) or ORF73. stop (triangles) MHV68. Significantly different groups were: control mock infected and control MHV68wt infected, control mock infected and HOIL-1 KO MHV68wt infected, control mock infected and HOIL-1 KO MHV68orf73. stop infected, HOIL-1 KO mock infected and control MHV68wt infected, HOIL-1 KO mock infected and HOIL-1 KO MHV68wt infected, control MHV68wt infected and control MHV68orf73. stop infected, control MHV68wt infected and HOIL-1 KO MHV68orf73. stop infected, HOIL-1 KO MHV68wt infected and control MHV68orf73. stop infected, HOIL-1 KO MHV68wt infected and HOIL-1 KO MHV68orf73. stop infected, control MHV68orf73. stop infected and HOIL-1 KO MHV68orf73. stop infected. *p ≤ 0. 0033; logrank Mantel–Cox test corrected for multiple comparisons. (C) Cytokine transcript levels in peritoneal cells from mock (circles) and MHV68-infected (squares) control (blue symbols) and HOIL-1 KO (red symbols) mice (28 days post-infection). (D) Induction of cytokine transcripts in peritoneal cells from mock (circles) and MHV68-infected (squares) control (blue symbols) and HOIL-1 KO (red symbols) mice (28 days) 3 hr after infection with 105 Listeria. Each symbol represents an individual mouse. For (C) and (D), *p ≤ 0. 05, **p ≤ 0. 01, ***p ≤ 0. 001, ****p ≤ 0. 0001. Statistical analyses were performed using one-way ANOVA. (E) Survival of control (blue symbols) and Il6−/− (purple symbols) mice challenged with 106 CFU Listeria 28 days following mock infection (circles) or infection with 106 PFU MHV68 (squares). *p ≤ 0. 0083; logrank Mantel–Cox test corrected for multiple comparisons. (F) Survival of control (blue symbols), Caspase-1; Caspase-11 (orange symbols) and Caspase-1 (green symbols) –deficient mice challenged with 106 CFU Listeria 28 days following mock infection (circles) or infection with 106 PFU MHV68 (squares). *p ≤ 0. 0033; logrank Mantel–Cox test corrected for multiple comparisons. DOI: http: //dx. doi. org/10. 7554/eLife. 04494. 01910. 7554/eLife. 04494. 020Figure 5—figure supplement 1. MHV68 latency-induced cross-protection is maintained for at least 6 months. Survival of control (blue symbols) and HOIL-1 KO (red symbols) mice challenged with 106 CFU Listeria 6 months after mock infection (circles) or infection with 106 PFU MHV68 (squares). Statistical analyses were performed by logrank Mantel–Cox test corrected for multiple comparisons, with p ≤ 0. 0083 considered significant. Control mock vs HOIL-1 KO mock, p = 0. 1824; control mock vs control MHV68 infected, p = 0. 0408; control mock vs HOIL-1 KO MHV68 infected, p = 0. 0070; HOIL-1 KO mock vs control MHV68 infected, p = 0. 0028; HOIL-1 KO mock vs HOIL-1 KO MHV68 infected, p = 0. 0003; control MHV68 infected vs HOIL-1 KO MHV68 infected, p = 0. 9303. DOI: http: //dx. doi. org/10. 7554/eLife. 04494. 02010. 7554/eLife. 04494. 021Figure 5—figure supplement 2. MHV68 latency enhances the listericidal activity of peritoneal macrophages. Listeria CFU in control (blue symbols) and HOIL-1 KO (red symbols) ex vivo cultures of peritoneal macrophages from mock (circles) or MHV68-infected (32 days, squares) mice at 0 and 6 hr post-infection. Data are combined from two independent experiments. Each symbol represents an individual mouse analyzed in duplicate. Cells from the same mouse were used for both 0 hr and 6 hr time points. Statistical analyses were performed by one-way ANOVA with Holm-Sidak' s multiple comparison test for each time point. DOI: http: //dx. doi. org/10. 7554/eLife. 04494. 02110. 7554/eLife. 04494. 022Figure 5—figure supplement 3. Il6−/− mice have increased susceptibility to Listeria infection. Survival of control (blue circles) and Il6−/− (purple squares) mice following i. p. inoculation with 105 CFU Listeria. *p ≤ 0. 0083; logrank Mantel–Cox test corrected for multiple comparisons. DOI: http: //dx. doi. org/10. 7554/eLife. 04494. 022 To determine whether MHV68 latency rescued pro-inflammatory cytokine induction by HOIL-1 KO mice following Listeria infection, we quantitated cytokine transcripts in peritoneal cells from latently infected mice before (Figure 5C) and 3 hr after (Figure 5D) infection with Listeria. As predicted from the cytokine levels in the serum, MHV68 latency resulted in small but significant increases in Tnf, Il6 and Il12b transcripts prior to Listeria challenge (Figure 5C). While MHV68 latency did not rescue the induction of Tnf or Il6 transcripts in HOIL-1 KO mice following infection with Listeria, Il12b transcript levels were increased approximately twofold, and were comparable to levels in control mice. More significantly, Ifng and Nos2 (encoding iNOS) transcripts were elevated approximately 200-fold and 1000-fold, respectively, in latently infected control and HOIL-1 KO mice (Figure 5C), and further induced by 3 hr after infection with Listeria (Figure 5D). Ifng transcript levels were significantly higher in latently infected HOIL-1 KO mice following infection with Listeria than in control mice. These data suggest that MHV68 latency by-passes the requirement for TNFα and IL-6 during early Listeria infection by enhancing the induction of IFNγ and downstream effector molecules important for controlling Listeria infection. To test whether peritoneal macrophages from latently infected HOIL-1 KO mice had an increased capacity to kill Listeria, we explanted peritoneal macrophages from mock or latently infected mice, infected them with Listeria, killed extracellular bacteria with gentamycin treatment, and compared the number of CFU at 6 hr to the number of CFU at the beginning of the experiment. As expected, cells from mock infected control mice exhibited mild listericidal activity and cells from latently infected control mice had an enhanced ability to kill Listeria (Figure 5—figure supplement 2, (Barton et al. , 2007) ). Macrophages from mock-infected HOIL-1 KO mice had a slightly impaired ability to control Listeria infection. However, MHV68 latency in HOIL-1 KO mice enhanced the ability of macrophages to kill Listeria, generating a capacity to kill similar to that observed with macrophages from control mice. Together, these data suggest that MHV68 latency induces an environment that enhances the ability of HOIL-1-deficient cells to kill and respond to Listeria. To determine whether the viral complementation of immunodeficiency was unique to HOIL-1, we latently infected IL-6, Caspase-1-deficient and Caspase-1; Caspase-11-double-deficient mice (Kayagaki et al. , 2013), which survive MHV68 infection but are all highly susceptible to Listeria infection (Figure 5—figure supplement 3) (Kopf et al. , 1994; Sarawar et al. , 1998; Edelson and Unanue, 2002; Tsuji et al. , 2004; Sauer et al. , 2011). IL-6, Caspase-1 and Caspase-1; Caspase-11-deficient mice were also protected from lethality following Listeria infection by chronic MHV68 infection (Figure 5E, F). These data indicate that the capacity of chronic MHV68 to reverse a significant immunodeficiency is not restricted to mutations in Rbck1 (Hoil1), and suggest that chronic viral infections may alter phenotypes of many host allelic variants.
We report that HOIL-1 is essential during infection with Listeria, T. gondii and C. rodentium, but not with MHV68 or M. tuberculosis in mice. Expression of HOIL-1 was critical in innate, hematopoietic-derived cells during Listeria infection in vivo. The requirement of HOIL-1 for the induction of protective inflammatory cytokines, TNFα, IL-6 and IL-12, following infection of macrophages with Listeria in vitro is consistent with reports that LUBAC is required for efficient NF-κB activation following TLR engagement (Zak et al. , 2011; Boisson et al. , 2012), but not with a recent report that NF-κB activation following stimulation of TLR4 and TNF-R1 on macrophages by LPS and TNFα, respectively, is unaffected by HOIL-1 deficiency (Rodgers et al. , 2014). These apparently conflicting results suggest that HOIL-1 and LUBAC may not only have cell-type specific functions (Boisson et al. , 2012; Rodgers et al. , 2014), but also stimulus-specific roles that vary between different cell types. This may be further highlighted by the excessive inflammatory response and enhanced control of MHV68 and M. tuberculosis infection—two pathogens that also infect macrophages—by HOIL-1-deficient mice. It has been proposed that low levels of viral reactivation during MHV68 latency are responsible for the low level of constant immune activation and cytokine production (Barton et al. , 2007). It is paradoxical, then, that reactivation is almost undetectable in HOIL-1-deficient animals, and yet their inflammatory response is elevated. Since the negative regulator of NF-κB signaling, A20, binds to linear ubiquitin chains (Tokunaga et al. , 2012; Verhelst et al. , 2012), HOIL-1/LUBAC may also be important for recruiting A20 to receptor signaling complexes to turn off signaling. Therefore, receptor signaling may be sustained in cells in HOIL-1 KO mice, resulting in the increase in TNFα, IL-6 and IL-12, and ultimately IFNγ protein, observed in the serum of chronically infected mice. Decreased viral reactivation may be the result of this increased IFNγ, the inability of latently infected cells to response to a stimulus of reactivation or a combination thereof. It is unclear why HOIL-1 KO mice are extremely susceptible to some pathogens, yet control other infections remarkably well. This might be due to HOIL-1/LUBAC having differential roles in transducing signals from different immune sensors stimulated by different infections. Other possibilities include a differential requirement for the innate immune system to hold the acute infection in check while an adaptive response is being generated, the speed at which the pathogen replicates, the tissues that it damages, and whether pathology during acute infection is mostly immune- or pathogen-derived. Further studies will be required to address these possibilities. We further show that chronic infection with MHV68 rescued HOIL-1, IL-6, Caspase-1 and Caspase-1; Caspase-11-deficient mice from lethal Listeria infection, thereby masking the genetic immunodeficiency observed in MHV68-negative mice. As reported previously (Barton et al. , 2007), MHV68 latency was associated with increased basal levels of pro-inflammatory cytokines TNFα, IL-6, IL-12 and IFNγ in the serum of wild-type animals, which were further increased in HOIL-1 KO mice. These cytokines appear to increase the activation status of the innate immune system such that their induction following Listeria infection is no longer as important as would be the case in a naïve mouse. Indeed, MHV68 infection did not enhance the transcription of Tnf, and only marginally enhanced the transcription of Il6 and Il12b even in control mice following Listeria infection, and did not rescue the defect in Tnf and Il6 induction observed in HOIL-1 KO mice. Conversely, transcription of Ifng and the effector molecule, iNOS (encoded by Nos2), was elevated in cells from latently infected mice and enhanced substantially by both HOIL-1 KO and control animals very rapidly following infection with Listeria. Macrophages require priming with IFNγ to induce IL-12 in response to Listeria infection. In a latently infected animal, IFNγ is already present and so IL-12 and additional IFNγ may be induced more rapidly. Furthermore, as reported previously for wild-type mice (Barton et al. , 2007), MHV68 latency enhanced the ability of peritoneal macrophages from control and HOIL-1 KO mice to kill Listeria. Together, these data suggest that the constant presence of low levels of IFNγ driven by latent virus infection results in an increase in the basal expression levels of downstream effector molecules and the priming of cells for the enhanced immediate killing of Listeria upon infection, as well as for a more rapid further induction of IFNγ and its effector molecules in response to the bacterial challenge. In this sense, chronic virus infection sets the level of innate immunity to subsequent infection. HOIL-1 KO mice bred in a high grade barrier facility failed to exhibit certain phenotypes of HOIL-1 deficient patients, specifically by not exhibiting baseline hyper-inflammation and by displaying a striking immunodeficiency out of proportion to that observed in some humans with bi-allelic mutations in RBCK1 (HOIL1). Most humans are infected life-long with multiple herpesviruses (Virgin et al. , 2009; Virgin, 2014), and many also carry other chronic or latent infections such as tuberculosis. Importantly, we observed complementation of immunodeficiency to Listeria by chronic herpesvirus infection in four different strains of immunodeficient mice, revealing virus infection as one possible environmental factor that might alter the genotype-phenotype relationship for patients with mutations in immune system genes. HOIL-1 KO mice chronically infected with either a herpesvirus or M. tuberculosis also exhibited increases in some of the same cytokines reported in the serum of HOIL-1 deficient humans. At least three of the HOIL-1 mutant patients were infected with at least one herpesvirus (Boisson et al. , 2012), and it is likely that other chronic infections were present. However, in the absence of data regarding the complete infection status of the HOIL-1 mutant patients, the relevance of the mouse studies to the human phenotypes is unclear. Nevertheless, perhaps the presence of the virome, and potentially variations in the virome or other chronic infections between people, confers significant phenotypic variation by complementing mutations in host genes responsible for innate immunity (Virgin, 2014). The genes involved in immunity and inflammation are the most rapidly evolving in the mammalian genome (Lindblad-Toh et al. , 2011; Casanova et al. , 2013; Quintana-Murci and Clark, 2013). Survival from infection requires a trade-off between alleles that promote or limit inflammation to balance immunity vs immunopathology. We speculate that the virome or other chronic infections hide or enhance the effects of genetic variations in immune responsiveness by complementing chromosomal variations in immune response genes. As the nature of the virome changes in persons growing up in different cultural and economic environments, it is possible that the immunophenotype of the host changes, and the beneficial or deleterious effects of existing genetic variation are unmasked by removal of complementation provided by chronic virus infection. It is also plausible that the striking auto-inflammation observed in humans with a variety of immune defects could be due to even well controlled herpesvirus infection alone or in combination with other chronic infections. Our data suggest that analysis of the metagenome, including the virome, may be of value in linking human phenotype and genotype (Virgin, 2014; Virgin and Todd, 2011). Recent rapid advances in sequencing and analysis of the metagenome will make integration of data from the virome into human genetic studies practical (Virgin, 2014).
HOIL-1 KO mice, with null mutations in the Rbck1 gene that encodes HOIL-1, have been described previously (Tokunaga et al. , 2009). C57BL/6J mice or HOIL-1 WT littermates were used as wild type controls. Rag1−/− mice were purchased from The Jackson Laboratory (Bar Harbor, ME) and bred to HOIL-1 KO mice. Il6−/− mice were purchased from The Jackson Laboratory. Caspase 1; Caspase 11-deficient mice with or without a Caspase 11 transgene were kindly provided by Vishva Dixit, Genentec, San Francisco USA. All mice were housed and bred at Washington University in Saint Louis in specific pathogen-free conditions in accordance with Federal and University guidelines and protocols were approved by the Animal Studies Committee of Washington University under protocol number 20140244. Mice were inoculated between 8 and 11 weeks of age. L. monocytogenes wild type strain EGD was used for this study. Listeria glycerol stocks were stored at −80 °C, and thawed and diluted into PBS for intraperitoneal (i. p.) injection into mice. To determine tissue burden, spleens and livers were homogenized in 10 ml PBS containing 0. 05% Triton X-100 and serial dilutions were plated on brain heart infusion agar plates. Listeria CFU were counted after overnight growth at 37°C. Small sections of spleen and liver were also fixed in 10% buffered formalin for histological analysis. The type II Prugniaud strain of T. gondii expressing a firefly luciferase (PRU-Fluc-GFP, provided by J. Boothroyd, Stanford University, Palo Alto, CA) (Saeij et al. , 2005) was used in all in vivo T. gondii experiments. Tachyzoites were grown by 2-day serial passage in human foreskin fibroblasts. For infections, freshly egressed parasites were filtered, counted, and injected i. p. into mice. Mice were with inoculated orally with 2 × 109 CFU C. rodentium strain DBS100 (ATCC, Manassas, VA) from a fresh culture and monitored for morbidity and mortality. MHV68 WUMS (ATCC VR1465), MHV68 ORF73. stop and γHV68 M3-Fluc were passaged and titered by plaque assay on NIH 3T12 cells. Virus stocks were stored at −80 °C, and thawed and diluted into PBS for i. p. or intranasal (i. n.) inoculation of mice. For experiments involving MHV68 ORF73. stop, i. n. inoculation with 5 × 104 CFU was performed due to the low titer of the virus stock. To determine γHV68 titers in tissues, organs were placed in 1 ml of complete DMEM and frozen at −80°C. Samples were thawed prior to disruption with silica beads and virus titration by plaque assay. Before infection, exponentially replicating M. tuberculosis Erdman strain bacteria were washed in PBS + 0. 05% Tween 80, and sonicated to disperse clumps. Mice were exposed to 8 × 107 CFU of M. tuberculosis in an Inhalation Exposure System (Glas-Col, Terre Haute, IN), which delivers ∼100 bacteria to the lung per animal. After 24 hr post infection, two mice per group were sacrificed, and lungs were harvested to determine infection efficiency, which was about 100 CFU/lung/mouse. Experimental mice were sacrificed 70 days after infection, and lungs and spleen were harvested for CFU, and serum was collected for cytokine analysis. Bacterial burdens were determined by plating serial dilutions of lung and spleen homogenates onto 7H10 agar plates and incubated at 37°C in 5% CO2 for 3 weeks prior to counting colonies. MHV68 reactivation from latency and preformed virus was assayed as described previously (Weck et al. , 1996). Briefly, peritoneal exudate cells were plated in twofold serial dilutions (24-wells per dilution) onto permissive mouse embryonic fibroblast (MEF) monolayers and scored for cytopathic effect (CPE) 3 weeks later. Reactivation of lytic virus from a peritoneal cell leads to complete CPE of the MEF monolayer. To measure preformed infectious virus in the sample, parallel samples of cells were mechanically disrupted to kill the cells but keep any infectious virus intact. These samples were plated and scored as described above. Using the Poisson distribution, CPE in 63. 2% of wells indicates that one reactivation event is likely to have occurred per well, and is used to determine the frequency of reactivating cells in the sample. To determine the frequency of cells harboring viral genome, peritoneal cells were assayed by nested PCR for viral genome as described previously (Weck et al. , 1999). The detection of PCR product in 63. 2% of wells indicates that one genome was present per well. Primary bone marrow-derived macrophages were prepared as described previously (Hwang et al. , 2012). Briefly, bone marrow was extracted from mouse femurs and allowed to differentiate in DMEM containing 10% FBS, 10% CMG14-12 cell-conditioned media as a source of M-CSF (Takeshita et al. , 2000), 5% horse serum, 1 mM sodium pyruvate and 2 mM L-Glutamine for 7 days. For cytokine and transcript analyses following Listeria infection of macrophages, adherent cells were scraped and seeded in tissue culture-treated plates in the absence of M-CSF. After 3 days, macrophages were infected with 106/ml Listeria in the presence or absence of 100 U/ml IFNγ. 2 hr post-infection, 50 U/ml Penicillin and 50 μg/ml streptomycin were added to kill the Listeria. Cell supernatants were harvested at indicated times and frozen at −80 °C prior to cytokine analysis. Cells were lyzed in TRI-Reagent for RNA extraction. For Listeria growth/killing assays, macrophages were seeded in non-tissue culture treated dishes in the absence of M-CSF. After 1 day, cells were treated with 300 µ/ml IFNγ or untreated, and 48 hr later scraped replated on sterile coverslips. After 3 hr, cells were infected with 105/ml Listeria from an overnight standing culture and centifuged to synchronize the infection. 50 μg/ml gentamycin was added after 30 min to kill extracellular bacteria. At the indicated times, coverslips were washed with warm PBS, then lyzed in 10 ml cold water to release the bacteria. Serial dilutions were plated on brain heart infusion agar plates, and Listeria CFU were counted after overnight growth at 37°C. For MHV68 growth analysis, adherent cells were scraped and seeded in tissue culture-treated plates in the presence of M-CSF. After 2 days, macrophages were treated with 0. 1 U/ml IFNγ or untreated, and 12 hr later infected with MHV68 at a multiplicity of infection (MOI) of 0. 05 for 1 hr with occasional rocking at 37°C and 5% CO2. Cells were washed once with medium and incubated in DMEM supplemented with 10% FBS and 2 mM L-glutamine (with or without 0. 1 U/ml IFNγ) for the indicated period of time at 37°C and 5% CO2, before being frozen at −80 °C. Virus titers were determined by plaque assay following two freeze–thaw cycles. Cells were flushed from the peritoneum of mice that had been mock infected or infected with MHV68 for 32 days with ice cold DMEM containing 10% FBS and 2 mM L-Glutamine. 5 × 105 cells were plated on glass coverslips in 24 well plates in duplicate wells per timepoint and allowed to adhere overnight. Non-adherent cells were washed away with warm medium, and the remaining cells were infected with 105 CFU Listeria from a overnight standing culture by spinocculation at 600×g for 10 min at room temperature, and then incubated at 37 °C and 5% CO2. 50 μg/ml gentamycin was added after 30 min to kill extracellular bacteria. Coverslips were washed in warm PBS prior to hypotonic lysis of the cells in ice cold water to release the bacteria. Serial dilutions were plated on brain heart infusion agar plates, and Listeria CFU were counted after overnight growth at 37°C. Recipient mice were exposed to 1200 rad of whole body irradiation, and injected intravenously with 10 million whole bone marrow cells from donor mice. Mice were allowed to reconstitute for 8 to 10 weeks before Listeria challenge. Mice were bled at 7 weeks post-irradiation to determine percent chimerism. Genomic DNA was isolated from peripheral blood and analyzed by quantitative real-time PCR (qRT-PCR) for the presence of Rbck1/Hoil1 intron 7 (in control cells; 5′-ATG CTG GAG TAG AGG CTG GA-3′ and 5′-TGA CTG CTG CTT GGA GAG TG-3′), or the neomycin-resistance cassette (in HOIL-1 KO cells; 5′-CAA GAT GGA TTG CAC GCA GG-3′ and 5′-GCA GCC GAT TGT CTG TTG TG-3′). Rag2 was used as a normalization control (5′-GGG AGG ACA CTC ACT TGC CAG TA-3′ and 5′-AGT CAG GAG TCT CCA TCT CAC TGA-3′). Imaging was performed as described previously (Saeij et al. , 2005). Briefly, mice were injected i. p. with 150 mg/kg D-Luciferin (Biosynth AG, Switzerland) and allowed to remain active for 5 min. Animals were subsequently anesthetized with 2% isoflurane for 5 min and then imaged with a Xenogen IVIS 200 machine (Caliper Life Sciences, Hopkinton, MA). Data were analyzed using Living Image software (Caliper Life Sciences). Peritoneal exudate cells were harvested by peritoneal lavage with 10 ml ice cold FACS buffer (PBS supplemented with 2% FBS and 50 U/ml Penicillin and 50 µg/ml Streptomycin). Splenocytes were isolated by filtering through two 100 μm cell strainers into 10 ml ice cold FACS buffer. Residual red blood cells were lysed with Red Blood Cell lysis buffer (Sigma, St Louis, MO), counted and stained for flow cytometry. Cells were incubated with FACS buffer plus 1% rat serum, 1% hamster serum and 1% Fc-block for 15 min. Surface staining was performed for 30 min at room temperature. Cells were then washed and fixed with 2% formaldehyde. Cells were analyzed on an LSRII or LSR Fortessa flow cytometer (BD, Franklin Lakes, NJ) and the data were analyzed using FlowJo software (Tree Star, Inc, Ashland, OR). The following antibodies were used: anti-CD3e (clone 145-2C11, Biolegend, San Diego, CA), anti-CD4 (clone RM4-5, BD Pharmingen), anti-CD8 (clone 53-6. 7, Biolegend), anti-IgM (clone II/41, BD Pharmingen), anti-CD19 (clone 6D5, Biolegend), anti-NK1. 1 (clone PK136, BD Pharmingen), anti-NKp46 (clone 29A1. 4, eBioscience, San Diego, CA), anti-Ly6C (clone HK1. 4, Biolegend), anti-Ly6G (clone 1A8, Biolgend, anti-CD11b (clone M1/70, BD Pharmingen), anti-F4/80 (clone BM8, Biolegend). Cytokines in mouse serum and cell supernatants were quantitated using a custom Procarta Immunoassay Kit (Affymetrix, Santa Clara, CA) and analyzed on a Bio-Plex 200 System (BioRad, Hercules, CA) or by ELISA (BD, Franklin Lakes, NJ), respectively, according to the manufacturers’ instructions. Spleen sections were homogenized, and macrophages were lysed in TRI-Reagent (Sigma), and processed according to the manufacturer' s instructions to isolate total RNA. RNA was isolated from peritoneal cells using RNeasy mini kit (Qiagen, Netherlands). RNA samples were treated with Turbo DNA-free DNase (Ambion, Austin, TX) prior to first strand cDNA synthesis with ImProm-II (Promega, Madison, WI) and random hexamer primers. Quantitative PCR was performed on a StepOnePlus machine using Power SYBR Green master mix (Applied Biosystems, Waltham, MA) and primers specific for ribosomal protein S29 (Rps29; 5′-AGC AGC TCT ACT GGA GTC ACC-3′ and 5′-AGG TCG CTT AGT CCA ACT TAA TG-3′), Rbck1/Hoil-1 (5′-ATT CGG CGG AAT GGA GAC GG-3′ and 5′-CTG GTT GGT CCT GGG CTT CG-3′), Trib3 (5′-CAC ACT GCC ACA AGC ACG GG-3′ and 5′-CAC GCA GGC ATC TTC CAG G-3′), Tbc1d20 (5′-TGA GGG AGG GCT CCT GAC TG-3′ and 5′-AGC AGC ACT TGC TGG TAG TCC-3′), Il12b (5′-GCA CGG CAG CAG AAT AAA TAT GAG-3′ and 5′-TTC AAA GGC TTC ATC TGC AAG TTC-3′), Tnf (5′-GGG TGA TCG GTC CCC AAA GG-3′ and 5′- CTG AGT GTG AGG GTC TGG GC-3′), Il6 (5′-GCC AGA GTC CTT CAG AGA GAT ACA-3′ and 5′-CTT GGT CCT TAG CCA CTC CTT C-3′), Ifng (5′-ATG AAC GCT ACA CAC TGC ATC-3′ and 5′-CCA TCC TTT TGC CAG TTC CTC-3′) and iNos (Nos2) (5′-GTT CTC AGC CCA ACA ATA CAA GA-3′ and 5′-GTG GAC GGG TCG ATG TCA C-3′). Transcript levels were analyzed using the ΔΔCT method, with Rps29 as the reference gene. Tissues were fixed in 10% buffered formalin followed by 70% ethanol, paraffin embedded, sectioned and stained with Periodic acid-Schiff (PAS). Alanine aminotransferase and aspartate aminotransferase were measured on a Liasys 330 (AMS Diagnostics, Weston, FL), complete blood counts were measured on a Hemavet 1700 (Drew Scientific, Waterbury, CT), and white blood cell differential counts were performed by Washington University Division of Comparative Medicine Animal Diagnostic Laboratory staff. Statistical significance was determined using GraphPad Prism software. The specific tests performed are noted in the figure legends. | The immune system protects an individual from invading bacteria, viruses and parasites, as well as malfunctioning or cancerous host cells. However, some people inherit genetic defects that cause part of the immune system to be missing or to not work properly. This is called a genetic immunodeficiency, and puts individuals at a higher risk of infection and disease. The symptoms of immunodeficiencies can vary substantially between individuals, even when they have defects in the same gene. For example, only some of the individuals who have defects in both of their copies of a gene called HOIL-1—which has been linked to several roles in the body' s immune response—are reported to suffer from an altered susceptibility to bacterial infections and chronic (persistent) inflammation. Gaining a clear understanding of the possible factors that influence such variations in the symptoms of genetic immune deficiencies could help to speed up their diagnosis, as well as helping to develop more effective treatments. MacDuff et al. studied mice that had mutations in both copies of the mouse equivalent of the HOIL-1 gene. These mice, when raised in a clean barrier facility that reduces their exposure to viruses, were severely immunodeficient and died when infected by certain bacteria and parasites, including Listeria monocytogenes. However, they were able to tolerate infections with a herpesvirus or the bacterium that causes tuberculosis. The immunodeficiency to L. monocytogenes was linked to problems producing protective molecules called cytokines, which form a crucial part of the immune response. Unexpectedly, MacDuff et al. found that a chronic herpesvirus infection substantially protected these very immunodeficient animals from infection with Listeria monocytogenes, and the mice were able to efficiently produce protective cytokines. Mice with two other distinct genetic deficiencies that affect their immune system were also better able to survive otherwise lethal bacterial infections if they had a long-term herpesvirus infection. Macduff et al. suggest that the chronic herpesvirus infection stimulates the immune system, and so allows it to compensate for the lack of cytokine production associated with various immunodeficiencies, including those caused by mutations in the HOIL-1 gene. This suggests that the presence of viruses or other long-term infections may be responsible for some of the variability seen in the symptoms of different individuals with the same genetic immunodeficiency. This is an important concept since essentially all humans have life-long chronic infections from various herpesviruses, as well as other viruses that form the human virome. | Abstract
Introduction
Results
Discussion
Materials and methods | microbiology and infectious disease
immunology and inflammation | 2015 | Phenotypic complementation of genetic immunodeficiency by chronic herpesvirus infection | 17,726 | 585 |
Rapid and flexible interpretation of conflicting sensory inputs in the context of current goals is a critical component of cognitive control that is orchestrated by frontal cortex. The relative roles of distinct subregions within frontal cortex are poorly understood. To examine the dynamics underlying cognitive control across frontal regions, we took advantage of the spatiotemporal resolution of intracranial recordings in epilepsy patients while subjects resolved color-word conflict. We observed differential activity preceding the behavioral responses to conflict trials throughout frontal cortex; this activity was correlated with behavioral reaction times. These signals emerged first in anterior cingulate cortex (ACC) before dorsolateral prefrontal cortex (dlPFC), followed by medial frontal cortex (mFC) and then by orbitofrontal cortex (OFC). These results disassociate the frontal subregions based on their dynamics, and suggest a temporal hierarchy for cognitive control in human cortex.
Flexible control of cognitive processes is fundamental to daily activities, including the execution of goal-directed tasks according to stimulus inputs and context dependencies. An important case of cognitive control arises when input stimuli elicit conflicting responses and subjects must select the task-relevant response despite competition from an often stronger but task-irrelevant response (Miller, 2000; Miller and Cohen, 2001). A canonical example of this type of conflict is the Stroop task: subjects are asked to name the font color of a word where the semantic meaning conflicts with the color signal (e. g. the word' red' shown in green versus red). Such incongruent inputs lead to longer reaction times, attributed to weaker signals (color processing) that must be emphasized over the automatic processing of word information (Stroop, 1935). The Stroop task is frequently used in cognitive neuroscience and clinical psychology and forms the foundation for theories of cognitive control. Neurophysiological, neuroimaging, and lesion studies have ascribed a critical role in cognitive control to networks within frontal cortex (Miller, 2000; Miller and Cohen, 2001), yet the neural circuit dynamics and mechanisms responsible for orchestrating control processes remain poorly understood. Lesion studies (Cohen and Servan-Schreiber, 1992; Perrett, 1974), human neuroimaging measurements (Egner and Hirsch, 2005a; MacDonald, 2000), and macaque single unit recordings (Johnston et al. , 2007) implicate the dorsolateral prefrontal cortex (dlPFC) in providing top-down signals to bias processing in favor of the task-relevant stimuli (Botvinick et al. , 2001; Miller and Cohen, 2001). The medial frontal cortex (mFC) also participates in cognitive control, possibly in a conflict monitoring capacity (Botvinick et al. , 2001; Ridderinkhof et al. , 2004; Rushworth et al. , 2004). Recordings and lesions studies in the macaque anterior cingulate cortex (ACC) (Ito et al. , 2003; Nakamura et al. , 2005) suggest that ACC neurons are principally involved in monitoring for errors and making between-trial adjustments (Brown and Braver, 2005; Ito et al. , 2003; Johnston et al. , 2007; Rothé et al. , 2011) —an idea that has received support by a recent study in the human ACC (Sheth et al. , 2012). Recent work has also demonstrated that the supplementary motor area and the medial frontal cortex play an important role in monitoring for errors (Bonini et al. , 2014). An alternative and influential theoretical framework posits that the ACC monitors for potential conflicts and subsequently directs the dlPFC to engage control processes (Botvinick et al. , 2001; Shenhav et al. , 2013). Several human neuroimaging studies are consistent with this notion (Botvinick et al. , 1999; Kerns, 2006; Kerns et al. , 2004; MacDonald, 2000) but the relative contributions of dlPFC, mFC, and ACC to cognitive control remain a matter of debate (Aarts et al. , 2008; Cole et al. , 2009; Fellows and Farah, 2005; Mansouri et al. , 2007; Milham and Banich, 2005; Milham et al. , 2003; Rushworth et al. , 2004). Previously, some neuroimaging studies have suggested that these frontal cortex regions can be differentiated based on the presence or absence of conflict signals (MacDonald, 2000). The challenge in dissociating the relative roles of these regions during Stroop-like tasks is that increased task difficulty recruits a host of executive functions (attention, decision-making, uncertainty, cognitive control). These functions are associated with neural activity spanning tens to hundreds of milliseconds and the underlying dynamics are difficult to untangle with the low temporal resolution of existing neuroimaging techniques (Shenhav et al. , 2013). Human single neuron studies provide millisecond resolution but have focused on individual regions (Sheth et al. , 2012). We took advantage of the high spatiotemporal resolution of intracranial recordings in human epilepsy patients and the ability to record simultaneously from multiple regions to directly investigate the dynamics of conflict responses during cognitive control. We hypothesized that subregions of frontal cortex could be differentiated based on the temporal profile of their conflict responses. We recorded intracranial field potentials from 1397 electrodes in 15 subjects while they performed the Stroop task or a variation in which they were asked to read the word instead of focusing on its color. We observed conflict-selective activity throughout several regions in frontal cortex: ACC, mFC, dlPFC, and also orbitofrontal cortex (OFC). Several analyses link these signals to cognitive control. Neural responses increased for incongruent compared to congruent trials, and these signals correlated with behavioral reaction time, depended on the task, and exhibited adaptation over trials. We compared pairs of simultaneously recorded electrodes to disassociate these different regions based on the timing of these conflict responses rather than their presence or absence. Conflict responses emerged first in the ACC and subsequently emerged in dlPFC and mFC and finally in OFC. These observations propose a plausible flow of signals underlying cognitive control.
We focused on 469 electrodes located in areas within frontal lobe which have been previously implicated in executive function: medial frontal cortex (mFC, n = 111), orbitofrontal cortex (OFC, n = 156), dorsolateral prefrontal cortex (dlPFC, n = 168) and the anterior cingulate cortex (ACC, n = 34). We applied a non-parametric analysis of variance (ANOVA) to measure whether and when the physiological responses differed between congruent and incongruent trials. An electrode was considered conflict-selective if the F-statistic was greater than a significance threshold computed by a permutation test with P = 0. 001 for 50 consecutive milliseconds (Materials and methods). The latency was defined as the first time of this threshold-crossing. Figure 2 shows an example electrode from the left Anterior Cingulate Cortex that responded differentially between congruent and incongruent trials during the Stroop task. These signals were better aligned to the speech onset than to the stimulus onset, as shown in the response-aligned view (compare Figure 2A–C with Figure 2D–F). During the Stroop task, the response-aligned signals were significantly stronger for the incongruent (brown) trials compared to the congruent (black) trials (Figure 2D, P < 10–5, ANOVA), and were invariant to the particular word/color combinations (Figure 2G). Incongruent trials could be discriminated from congruent trials at a latency of 669 ± 31 ms (mean ± s. e. m.) before the onset of the response (Figure 2D). This conflict response was also specific to the Stroop task; there was a significant interaction between congruency and task (F = 13. 5, P = 0. 007, ANOVA). The same stimuli did not elicit differential activity during the Reading task (Figure 2F). We assessed the correlation between the neural signal strength and behavioral reaction times in single trials. The maximal gamma power during each incongruent trial (using the average gamma power yielded similar results) was positively correlated with the behavioral reaction times (Figure 2H, ρ = 0. 25, P = 0. 02). 10. 7554/eLife. 12352. 006Figure 2. Example electrode in left Anterior Cingulate Cortex. (A) Average gamma power signals aligned to the stimulus onset from an electrode during the Stroop task, for congruent (black) or incongruent (brown) stimuli. For display purposes only, we z-scored the gamma power by subtracting the average and dividing by the standard deviation of power during the baseline period (500 ms prior to stimulus onset). Shaded areas indicate s. e. m. The total number of trials for each condition is indicated in the upper right. (B) Single-trial data for congruent (left) and incongruent (right) trials. Each row is a trial, and the color indicates the z-scored gamma power (color scale on upper right). Trials are sorted by behavioral response time (black line). (C) Same as (A), but showing data from the Reading task. (D-F) Same as in A-C, but aligning the data to behavioral response time. Gamma power was better aligned to the behavioral response, and was stronger for incongruent compared to congruent trials. The dashed line indicates the response-aligned latency, defined as the first time point at which incongruent and congruent trials can be discriminated. (G) Signals elicited by each of the 9 possible stimulus combinations. (H) There was a correlation between the maximal z-scored gamma power and behavioral reaction times during incongruent trials (Pearson correlation coefficient = 0. 25, P = 0. 02, permutation test). Each point in this plot represents a single trial. DOI: http: //dx. doi. org/10. 7554/eLife. 12352. 00610. 7554/eLife. 12352. 007Figure 2—source data 1. Conflict-selective electrode data. DOI: http: //dx. doi. org/10. 7554/eLife. 12352. 00710. 7554/eLife. 12352. 008Figure 2—figure supplement 1. Example conflict-selective electrode in the right dorsolateral Prefrontal Cortex. Here we show a different conflict selective electrode, located in the dlPFC (format as in Figure 2). DOI: http: //dx. doi. org/10. 7554/eLife. 12352. 00810. 7554/eLife. 12352. 009Figure 2—figure supplement 2. Example conflict-selective electrode in the Orbitofrontal Cortex comparing responses in the Theta and Gamma Bands. (A-F) Responses in the theta power frequency band, z-scored. Same format as Figure 2—figure supplement 1. (G-L) Responses in the gamma power frequency band, z-scored. Same format as Figure 2—figure supplement 1. DOI: http: //dx. doi. org/10. 7554/eLife. 12352. 009 Any differences between congruent and incongruent trials in the stimulus-aligned analyses can be confounded by the reaction time differences; therefore, we focus subsequent analyses on the response-aligned signals. More example electrodes are shown in Figure 2—figure supplement 1 (dlPFC) and Figure 2—figure supplement 2 (OFC). Using the aforementioned criteria, we identified n = 51 conflict selective frontal cortex electrodes during the Stroop task, with contributions from 13 subjects (Supplementary files 2 and 3). These electrodes were distributed throughout different subregions within frontal cortex (Figure 3A). To evaluate whether random variation in the signals could give rise to apparent conflict-selective electrodes, we randomly shuffled the congruent/incongruent trial labels 10,000 times and applied the same statistical criteria (Materials and methods). Across our population, we found n = 4. 4 ± 0. 03 false positive electrodes (mean ± s. e. m. , out of 469 electrodes), which corresponds to a false discovery rate (FDR) of q = 0. 01, which is significantly less than our observation of n = 51 electrodes. The number of conflict-selective electrodes within each subregion was significantly greater than expected by chance (Figure 3B, P < 0. 01, all regions). We repeated the analyses during the Reading task. In contrast with the Stroop task, we only observed n = 3 conflict-selective frontal cortex electrodes during the Reading task (out of 469 electrodes), a number that is within the false positive rate. 10. 7554/eLife. 12352. 010Figure 3. Electrode locations. (A) Location of conflict-selective electrodes (black/gray) shown on a reference brain, with each region colored (Materials and methods). Electrodes from the right hemisphere were mapped to the left hemisphere for display purposes. For more detail, see Supplementary file 2. (B) Percent of total electrodes in each region that were selective for conflict. Chance levels were computed using a permutation test (black line). The number of observed electrodes was significantly above chance for all regions (P < 0. 01, permutation test, Materials and methods). DOI: http: //dx. doi. org/10. 7554/eLife. 12352. 01010. 7554/eLife. 12352. 011Figure 3—source data 1. Population gamma-power data. DOI: http: //dx. doi. org/10. 7554/eLife. 12352. 011 To account for within-subject and across-subject variation, we used a multilevel model (Aarts et al. , 2014) to conduct a group analysis of the physiological responses, with electrodes nested within subjects (Materials and methods). Across the population, we observed a significant interaction between the factors congruency and task on the gamma power (χ2=9. 2, P = 0. 002). Consistent with the single electrode examples, gamma power was greater for incongruent compared to congruent trials, but only during the Stroop task (Figure 4A, Stroop: P < 10–3, Reading: P = 0. 56). We computed the average response in each region (Figure 4B). Each electrode’s response was normalized by dividing the power during incongruent trials by the power in congruent trials (dividing the brown curve by the black curve in Figure 2), computing the logarithm and finally pooling within each region. The pooled responses in the OFC are visually less compelling (Figure 4B, bottom right subplot) due to the heterogeneity in the latency of the individual electrodes but the responses in the OFC were as vigorous as the ones in other areas (e. g. Figure 2—figure supplement 2). Similar conclusions were reached when plotting the pooled responses aligned to stimulus onset (Figure 4—figure supplement 3). 10. 7554/eLife. 12352. 012Figure 4. Gamma power in frontal cortex correlates with behavior. (A) Distribution of gamma power log-ratio (Incongruent/Congruent) for the Stroop task (blue) and Reading task (green). Bin size = 0. 05. Gamma power showed a significant interaction between Congruency and Task (P = 0. 002, multilevel model, Materials and methods). Power was larger for incongruent versus congruent trials during the Stroop task (P < 0. 001, n = 51 frontal cortex electrodes) but not during the Reading task (green, P = 0. 56). The statistical analyses directly compare the gamma power, we show the log-ratios here for display purposes only. (B) Normalized gamma power log-ratio averaged across electrodes from each of the four different frontal cortex regions during the Stroop task. We divided the power during incongruent trials by the power during congruent trials, then computed the log and finally averaged across electrodes. Data are aligned to the behavioral response onset (t=0). (C) Distribution of Pearson correlation coefficients between the maximal gamma power and behavioral reaction time during incongruent trials for n = 51 frontal cortex electrodes. These correlations were significantly positive (P < 10–5, sign-rank test). Bin size = 0. 1. (D) For incongruent trials, there was a significant interaction between trial history and task (P = 0. 03, multilevel model). Gamma power was larger for incongruent trials preceded by congruent trials (cI) compared to incongruent trials preceded by incongruent trials (iI), particularly during the Stroop task (blue, P = 0. 001), compared to the Reading task (green, P = 0. 72). Data beyond the range of the x-axis are shown in the first or last bins. (E) For congruent trials, there was no interaction between trial history and task (P = 0. 17, multilevel model). Gamma power was similar in congruent trials preceded by incongruent trials (iC) compared to congruent trials preceded by congruent trials (cC) during the Stroop task (blue, P = 0. 16) and during the Reading task (green, P = 0. 19). DOI: http: //dx. doi. org/10. 7554/eLife. 12352. 01210. 7554/eLife. 12352. 013Figure 4—source data 1. Population gamma-power data. DOI: http: //dx. doi. org/10. 7554/eLife. 12352. 01310. 7554/eLife. 12352. 014Figure 4—figure supplement 1. Theta and Beta band population results. (A) Distribution of theta power log-ratio (Incongruent/Congruent) for the Stroop task (blue) and Reading task (green). Bin size = 0. 05. P values in black denote interaction statistics whereas P values in blue and green denote the statistics for the Stroop and Reading tasks respectively. As discussed in Figure 4, the average log-ratios are presented here for display purposes only and the statistical tests are based on the raw power values. (B) Distribution of the gamma power log-ratio between incongruent trials preceded by congruent trials (cI) compared to incongruent trials preceded by incongruent trials (iI). (C) Distribution of the gamma power log-ratio between congruent trials preceded by incongruent trials (iC) compared to congruent trials preceded by congruent trials (cC). (D-F) Same as (A-C), but for power in the beta band. DOI: http: //dx. doi. org/10. 7554/eLife. 12352. 01410. 7554/eLife. 12352. 015Figure 4—figure supplement 2. Cross-frequency coupling analyses. For the anterior cingulate cortex electrode in Figure 2: (A) Phase-amplitude distribution during the Stroop task for the example electrode shown in Figure 2 (see Materials and methods for calculation of cross-frequency coupling). (B) The observed Modulation Index (MI, black arrow) is significantly greater than the surrogate distribution generated by adding a lag between the phase and amplitude measurements, demonstrating that the amplitude of the gamma band is strongly coupled to the phase of the theta band. (C) During the Stroop task, the difference in Modulation Index between congruent and incongruent trials (black arrow) was not significantly different from 0 (P = 0. 61). The null distribution (gray bars) was generated by randomly permuting the congruent and incongruent labels. Across the population of electrodes: (D) The percent of total electrodes in each region (Frontal cortex or non-frontal cortex) that had significant phase-amplitude coupling. Shown on the right is the percentage of the n = 51 conflict selective electrodes that showed significant coupling. (E) The MI of congruent compared to incongruent trials for all Frontal cortex electrodes (gray dots) and the subset that were conflict-selective in the gamma band (blue dots). For both groups, there was no significant difference in the MI between congruent and incongruent trials (Frontal Cortex, P = 0. 45; Conflict-selective, P = 0. 52; signed-rank test). For this comparison, the number of congruent and incongruent trials was equalized before computing the MI. DOI: http: //dx. doi. org/10. 7554/eLife. 12352. 01510. 7554/eLife. 12352. 016Figure 4—figure supplement 3. Stimulus-aligned population averages. Same as in Figure 4B, but data are aligned to the stimulus response onset (t=0). DOI: http: //dx. doi. org/10. 7554/eLife. 12352. 016 Several lines of evidence demonstrate a link between the neural signals described in the previous section and cognitive control: the neural signals correlated with reaction times, showed behavioral adaptation, and demonstrated error monitoring. As shown in previous studies, there was a wide distribution of behavioral reaction times (Figure 1B). Consistent with the example electrode in Figure 2, behavioral reaction times across the population correlated with the strength of the physiological signals, even after controlling for trial history (Figure 4C, P < 10–5, sign-rank test). The strength of these neural signals also revealed a neural correlate of the behavioral Gratton effect documented in Figure 1D: gamma power was greater in cI compared to iI trials (Figure 4D). Using the aforementioned multilevel model, we found a significant interaction between trial history (cI or iI) and task (χ2=4. 4, P = 0. 03). This Gratton effect was stronger in the Stroop task (P < 0. 001) than in the Reading task (P = 0. 72). These differences were not observed for cC versus iC trials, where the interaction was not significant (χ2=1. 9, P = 0. 17) (Figure 4E). This analysis was performed after removing stimulus repetition trials. The Gratton effect was present in all four frontal regions and there were no statistically significant differences in the strength of the effect across regions (F = 0. 25, P = 0. 86, ANOVA). To control for reaction time effects on these comparisons, we ran an analysis of covariance (ANCOVA) to test for a main effect of trial history on the gamma power with the behavioral reaction time as a covariate (Materials and methods). The neural Gratton effect during the Stroop task persisted under these controlled conditions (P = 0. 0002, multilevel model). We also explicitly ruled out reaction time differences by subsampling to match the reaction time distribution between conditions, with similar results (P = 0. 01, multilevel model). Together, these results suggest that the neural signals described here code for an internally perceived level of conflict that exhibits conflict adaptation and correlates with the across-trial variability in reaction times. The results presented above focus on the neural signals filtered within the gamma frequency band (70–120 Hz). We also examined the responses elicited in the broadband signals (1 to 100 Hz) as well as in the theta, (4 to 8 Hz), beta, (9 to 30 Hz), and low gamma (30–70 Hz) bands. No conflict selective responses were observed in the broadband signals or low gamma band. We found conflict-selective responses both in the theta and beta bands (see example in Figure 2—figure supplement 2a–f). Across theta and beta frequency bands, we also observed a significant interaction between Congruency and Task (theta: P < 10–5, beta: P < 10–4, multilevel model). Consistent with the results reported in the gamma frequency band, conflict responses in the theta and beta bands were more prominent during the Stroop task compared to the Reading task (Figure 4—figure supplement 1). In contrast to the results in the gamma band, power in the theta and beta bands decreased during incongruent trials. Furthermore, power in the theta and beta frequency bands was not correlated with reaction times (theta: P = 0. 43, beta: P = 0. 09, sign-rank test). In addition to separately examining the responses in different frequency bands, an important aspect of encoding of cognitive information is the relationship between signals across frequencies. In particular, several studies have demonstrated that the amplitude of the gamma band is coupled to the phase of slower oscillations in the theta band (Canolty et al. , 2006; Oehrn et al. , 2014; Tort et al. , 2008). We therefore examined the degree of cross-frequency coupling between the signals in the gamma and theta bands (Figure 4—figure supplement 2). Consistent with previous studies, we found that 50% of the electrodes demonstrated significant theta-gamma coupling. However, the strength of this coupling was not different between congruent and incongruent trials across the population of conflict-selective electrodes (P = 0. 52, sign-rank test). The conflict responses reported above are based on correct trials only. Yet, error monitoring has also been ascribed to frontal cortical circuits (Bonini et al. , 2014; Shenhav et al. , 2013; Yeung et al. , 2004). To investigate whether the same electrodes responding to conflict are also involved in successful error monitoring, we analyzed the neural signals during self-corrected trials. In these trials, subjects initially made an erroneous response and rapidly corrected themselves with the right answer. Given the high performance level of all subjects, the number of such trials is low. However, these trials are particularly interesting because we can be certain that there was successful error detection (as opposed to error trials without any self-correction). An example self-corrected trial from the ACC electrode shown previously is illustrated in Figure 5A. The subject initially made an incorrect response (green), which was rapidly followed with the correct response (red). Increased gamma power was observed after onset of the erroneous response. In contrast, the following corrected behavioral response exhibited no such post-response signal. Additionally, these error-monitoring signals were not observed in correct incongruent trials (Figure 2D), and were consistent across the n = 11 self-corrected trials for this subject (Figure 5B, P = 0. 001, signed rank test). Another example electrode is shown in Figure 5C–D. There were only two subjects contributing n = 7 conflict-signaling electrodes that had a sufficient number of self-correction trials (greater than five trials) for this analysis. For each electrode, we compared the difference in neural signals during the one-second post-response window between the initial error and the following self-correction. Of those n = 7 electrodes, n = 5 electrodes showed evidence of error monitoring (Figure 5E, P < 0. 05, sign-rank test). Although the number of electrodes and trials in this analysis is small, these results provide a direct correlate of error monitoring signals. Furthermore, these results highlight that the same electrodes that respond to conflict leading up to the behavioral response can also show post-response error monitoring. 10. 7554/eLife. 12352. 017Figure 5. Responses during self-corrected error trials. (A) An example self-correction trial from the ACC electrode in Figure 2 when the word Green colored in red was presented. The single trial gamma power is shown on top, with the speech waveform below. The dashed lines indicate the onset of the initially incorrect response (' green' ) and the following corrected response in bold (' no – red' ). Note the increased gamma power after an error response. (B) Average gamma power aligned to the onset of the initial error response (blue) and the onset of the corrected response (black) for n = 11 self-correction trials. Shaded areas indicate s. e. m. The post-response power was significantly greater after the error (P = 0. 001, signed-rank test). (C-D) Same as (A-B) for another example electrode in the dorsolateral prefrontal cortex. The post-response power was significantly greater after the error response (P = 0. 002, signed-rank test). (E) Across the n = 7 electrodes with n = 10 or greater self-correction trials, the z-scored gamma power during the initial error response was larger than during the corrected response. Electrodes with significant differences (P < 0. 05, signed-rank test) are colored black. Letters mark the examples in (A) and (C). DOI: http: //dx. doi. org/10. 7554/eLife. 12352. 01710. 7554/eLife. 12352. 018Figure 5—source data 1. Data for self-correcting trials. DOI: http: //dx. doi. org/10. 7554/eLife. 12352. 018 We observed conflict-selective responses in the anterior cingulate cortex, medial frontal cortex, dorsolateral prefrontal cortex and orbitofrontal cortex. To examine the dynamics of cognitive control orchestrating the transformation of conflicting visual signals to motor outputs, we compared, across those four regional groups, the latencies relative to behavioral response onset at which the congruent and incongruent trials could be discriminated. Comparing latencies across regions is difficult, especially across subjects with varying reaction times. For a controlled and direct comparison, we restricted the analysis to compute the latency differences between pairs of simultaneously recorded electrodes. This within-subject pairwise analysis had increased power to examine the relative dynamics between frontal lobe areas (Figure 6). The relative latencies were significantly different across the regions (P = 0. 01, permutation test, post-hoc testing was controlled for multiple comparisons using the Benjamin-Hochberg procedure, Materials and methods). Conflict responses in the ACC preceded those in all the other frontal lobe regions, followed 207 ± 40 ms later by dorsolateral prefrontal cortex and 388 ± 83 ms later by medial frontal cortex. Signals in orbitofrontal cortex emerged 319 ± 78 ms after dlPFC. This entire processing cascade took approximately 500 ms. For comparison, subjects’ behavioral reaction times to incongruent trials were 1105 ± 49 ms. The latency difference between ACC and dlPFC is based on 6 electrode pairs: one ACC electrode and six simultaneously recorded dlPFC electrodes. There was only one pair of simultaneous recordings between ACC and OFC and we do not report this value in Figure 6. The other region comparisons have contributions from multiple electrodes in multiple subjects (Supplementary file 3). These results suggest a temporal hierarchy of cognitive control mechanisms culminating in speech onset. 10. 7554/eLife. 12352. 019Figure 6. Latency Comparisons across regions. Latency differences between different regions computed from all pairs of simultaneously recorded electrodes. np denotes the number of electrode pairs. Because we only consider simultaneously recorded electrodes here, not all the electrodes modulated by conflict can be paired with any other electrode. Supplementary file 3 shows the number of electrodes modulated by conflict in each area and subject. There was only one electrode pair between ACC and OFC and therefore we do not show the latency difference between these two regions here. Significant latency differences (P < 0. 05, permutation test, Materials and methods) are shown in black, and non-significant differences in gray. ACC leads both mFC (P = 0. 001) and dlPFC (P = 0. 02), with OFC following dlPFC (P = 0. 009). DOI: http: //dx. doi. org/10. 7554/eLife. 12352. 01910. 7554/eLife. 12352. 020Figure 6—source data 1. Data for region latency comparisons. DOI: http: //dx. doi. org/10. 7554/eLife. 12352. 020
We used intracranial field potentials to measure the dynamics of conflict responses across frontal cortex leading up to the behavioral response in the Stroop task. Previous physiological and functional neuroimaging studies have documented the involvement of multiple of these frontal cortex areas in the Stroop or similar tasks (Botvinick et al. , 1999; Kolling et al. , 2012; MacDonald, 2000; Niendam et al. , 2012; Oehrn et al. , 2014; Sheth et al. , 2012). The intracranial field potential recordings reported here show conflict-selective signals in ACC (e. g. Figure 2), dlPFC (e. g. Figure 2—figure supplement 1), mFC (e. g. Figure 4B) and OFC (e. g. Figure 2—figure supplement 2). The mFC and dlPFC have been previously implicated in cognitive control, and these structures are extensively connected to the rest of frontal cortex areas (Ridderinkhof et al. , 2004). The role of the OFC in cognitive control during Stroop-like tasks has not been reported previously, possibly because of technical challenges in neuroimaging near this area (Weiskopf et al. , 2006). We presented several lines of evidence that demonstrate that these conflict-selective physiological signals are relevant for behavior during the Stroop task. Longer behavioral reaction times were correlated with greater gamma power on a trial-by-trial basis during the Stroop task but not during the Reading task, even after accounting for trial history and for differences between congruent and incongruent stimuli (Figure 2H, 4C). The same identical stimuli can elicit a range of behavioral reaction times and this internal degree of conflict can be captured, at least partly, by the strength of gamma power in frontal cortex in each trial. The neural correlates of behavioral adaptation (Gratton effect [Gratton et al. , 1992]) were observed in the ACC, consistent with prior studies based on human single neuron recordings (Sheth et al. , 2012), neuroimaging (Botvinick et al. , 1999; Kerns, 2006) and also in accordance with the behavioral effects of ACC resection (Sheth et al. , 2012). Conflict responses throughout the other frontal cortex regions also demonstrated the neural Gratton effect, suggesting a more distributed network involved in across-trial adaptation than previously hypothesized. The physiological responses in these areas were stronger in cI trials (incongruent trials that were preceded by congruent trials) than iI trials (Figure 4D). While the increased activity in cI trials compared to iI trials is consistent with neuroimaging studies (Botvinick et al. , 1999), single neuron recordings in a different Stroop-like task report the opposite relationship (iI > cI) (Sheth et al. , 2012). These differences point to potentially interesting distinctions between the activity of individual neurons and coarser population measures that warrant further investigation. Another discrepancy between neuroimaging studies and single unit recordings is the presence of conflict responses and error signals. Single unit recording in macaque ACC typically find error monitoring signals but not conflict-selective responses (Cole et al. , 2009; Emeric et al. , 2010; Ito et al. , 2003; Taylor et al. , 2006), see however (Ebitz and Platt, 2015), whereas human neuroimaging studies report both types of signals in ACC. There has been significant debate concerning whether action monitoring and conflict detection represent distinct processes (Carter et al. , 1998; Carter et al. , 2000; Nee et al. , 2011; Swick and Turken, 2002). Because both processes may co-occur on the same trials, high temporal resolution is required to disassociate the two computations. A recent human intracranial study has found error signals in supplementary motor area and medial frontal cortex (Bonini et al. , 2014), and a human single unit study reported conflict signals in ACC (Sheth et al. , 2012). The current work demonstrates the coexistence of both error signals and conflict signals. The analysis of the few self-correction trials in our data suggests that the same areas responsible for pre-behavioral conflict signals can also produce post-behavioral response error-monitoring signals (Figure 5). In addition, the relative timing of the conflict and error signals surrounding the neural responses confirms computational predictions based on a connectionist architecture to explain the mechanisms of conflict (Yeung et al. , 2004) and scalp EEG studies (Hughes and Yeung, 2011). These results are consistent with computational models suggesting that these signals may represent a general error-likelihood prediction, of which conflict and error detection are special cases (Brown and Braver, 2005). It has been suggested that ACC and supplementary eye field neurons in macaque monkeys respond to specific stimulus and/or behavioral combinations but are not directly modulated by conflict (Cole et al. , 2009; Nakamura et al. , 2005). At the level of the intracranial field potentials reported here, the modulation of conflict trials observed in the four frontal cortex regions could not be ascribed to specific stimulus or behavioral responses (e. g. Figure 2G) and were also task dependent (compare Figure 2A versus 2C). In these patients, we did not have access to single neuron responses and we therefore cannot rule out the possibility that individual neurons show distinct patterns of responses that are averaged out at the field potential level. Besides the high gamma band, we also observed conflict responses in the beta and theta bands, but not the low gamma band (e. g. Figure 4—figure supplement 1). Previous work has suggested differential roles for distinct oscillatory components of the local field potential (Cavanagh and Frank, 2014; Kahana et al. , 2001; Ullsperger et al. , 2014; von Stein and Sarnthein, 2000). There were clear differences in the type of information conveyed by distinct frequencies components. Lack of significant correlations with reaction time in the theta and beta bands suggests that the gamma band better captures the behavior. Additionally, conflict responses were characterized by increased power in the gamma band, but decreased power in the theta and beta bands (Figure 4—figure supplement 1). Previous scalp EEG recordings (Cavanagh and Frank, 2014; Ullsperger et al. , 2014; van Driel et al. , 2015) have demonstrated that conflict and/or error trials elicit increased theta power, suggesting potentially interesting differences in how theta is captured across spatial scales. We also observed a decrease in beta power, which is consistent with previous studies that correlate frontal cortex activation with desynchronization in the beta band and increased synchronization in the gamma bands (Crone et al. , 1998a; Crone et al. , 1998b). Differences across tasks, recording methods, and targeted regions should be interpreted with caution. The roles of different oscillatory components in neocortex are not clearly understood. One possibility is that lower frequency bands reflect the summed dendritic input of the nearby neural population (Logothetis et al. , 2001; Mitzdorf, 1987) and can act as channels for communication (Cavanagh and Frank, 2014), whereas higher frequency bands represent the population spiking rate (Buzsaki et al. , 2012; Ray and Maunsell, 2011). Along these lines, we speculate that the theta desynchronization we observe could reflect a reduction of inputs, leading to inhibition of the prepotent but erroneous response. While we observed conflict responses throughout frontal cortex, the spatiotemporal resolution of our intracranial recordings allowed us to separate regions by the latency at which conflict-selective responses emerge with respect to speech onset. By comparing pairs of simultaneously recorded electrodes, we found that conflict responses in the ACC lead the dlPFC by ~200 ms. Medial frontal cortex is anatomically close and extensively connected to the ACC, and the two regions are often grouped together (Cavanagh et al. , 2009; Ridderinkhof et al. , 2004). Yet, conflict responses in the mFC trail the ACC by hundreds of milliseconds, suggesting an important distinction between the two regions (Rushworth et al. , 2004). The relative latency measurements place the OFC at the bottom of this cascade. The hierarchical cascade of processes described here is consistent with predictions from mechanistic models of cognitive control (e. g. see Figure 2 in Shenhav et al, Neuron 2013). In particular, stimulus related signals are evident along the ventral visual stream early on and feed onto frontal cortex, where we find that ACC activity precedes activity in other frontal regions, followed by dlPFC, and finally mFC, and OFC. Since the local field potential pools over many neurons, latency measures can be influenced by a variety of factors, such as the proportion of neurons selective for conflict and their laminar organization. Yet, at least in the ACC, the temporal profile of conflict responses we observed is similar to responses from human single unit recordings (Sheth et al. , 2012). The relatively long delays between regions are also particularly intriguing. There are monosynaptic connections that link these four regions within frontal cortex and yet, it takes 100–200 ms to detect the relative activation between these areas (Figure 6). Daily decisions require integration of different goals, contexts, input signals, and the consequences of the resulting actions. The current study provides initial steps to elucidate not only which brain areas participate in cognitive control on a trial-by-trial basis but also their relative interactions and differential roles. The relative latency measurements and correlations between neural activity and reaction time provide a framework to constrain theories of cognitive control, and propose a plausible flow of conflict responses through frontal cortex.
Subjects were 15 patients (10 male, Ages 10–50, Supplementary file 1) with pharmacologically intractable epilepsy treated at Children’s Hospital Boston (CHB), Johns Hopkins Medical Institution (JHMI), Brigham and Women’s Hospital (BWH), or Taipei Veterans General Hospital (TVGH). These subjects were implanted with intracranial electrodes in frontal cortex for clinical purposes. Five other subjects participated in this task but they were excluded from the analyses because they did not have any electrodes in frontal cortex. All studies were approved by each hospital’s institutional review boards and were carried out with the subjects’ informed consent. Subjects were implanted with 2 mm diameter intracranial subdural electrodes (Ad-Tech, Racine, WI, USA) that were arranged into grids or strips with 1 cm separation. Electrode locations were determined by clinical considerations. There were 1397 electrodes (15 subjects). Sampling rates ranged from 256 Hz to 1000 Hz depending on the equipment at each institution: CHB (XLTEK, Oakville, ON, Canada), BWH (Bio-Logic, Knoxville, TN, USA), JHMI (Nihon Kohden, Tokyo, Japan), and TVGH (Natus, San Carlos, CA). All the data were collected during periods without any seizure events or immediately following any seizures. A schematic of the task is shown in Figure 1. After 500 ms of fixation, subjects were presented with a word stimulus for 2 s. The stimulus presentation was 3 s in two subjects. Stimuli were one of three words (Red, Blue, Green) presented in the subjects’ primary language (CHB, BWH, JHMI: English; TVGH: Mandarin) either in red, blue, or green font color. Stimuli subtended approximately 5 degrees of visual angle and were centered on the screen. Trials were either congruent (C), where the font color matched the word, or incongruent (I), where the font color conflicted with the word. The order of congruent and incongruent trials was randomized. Approximately 40% of the trials were incongruent trials. Within congruent trials and within incongruent trials all color-word combinations were counter balanced and randomly interleaved. Subjects were asked to either name the color (Stroop task) or read the word (Reading task) within the time limit imposed by the stimulus presentation time. Each block contained 18 trials, and the two tasks were completed in separate blocks. Most subjects completed 18 blocks of the Stroop task and 9 blocks of the Reading task (Supplementary file 1). Audio was recorded using a microphone at 8192 Hz sampling rate. No correct/incorrect feedback was provided. Electrodes were localized by co-registering the preoperative magnetic resonance imaging (MRI) with the postoperative computer tomography (CT) (Destrieux et al. , 2010; Liu et al. , 2009). In 4 subjects without a postoperative CT, electrodes were localized using intraoperative photographs and preoperative MRI. For each subject, the brain surface was reconstructed from the MRI and then assigned to one of 75 regions by Freesurfer. Depth electrodes were assigned to either a subcortical structure or to gyri/sulci. We focused on those electrodes in four frontal cortex regions (ACC: anterior and middle-anterior cingulate gyrus, mFC: superior frontal gyrus, dlPFC: middle frontal gyrus, and OFC: orbitofrontal gyrus). To determine the behavioral reaction time for each trial, the short-time energy was computed from the audio recordings. For an audio signal x (t), the short-time energy E (t) is defined as: E (t) =∑m=0m=T[x (m) w (t−m) ]2, where T is the length of the recording and w (t) is a 300-point Hamming window (~40 ms). Speech onset was defined as the first time when the energy crossed a threshold set as 1 standard deviation above the baseline. Only trials where the subject gave a single verbal response and the speech onset could be identified were considered correct trials. Unless otherwise noted, analyses in this manuscript used correct trials only. Electrodes with significant spectral noise were excluded from analysis (n = 25 out of 1397 total electrodes). For each electrode, a notch filter was applied at 60 Hz, and the common average reference computed from all channels was subtracted. Power in the theta (4–8 Hz), beta (9–30 Hz), and high-gamma band (70–120 Hz) was extracted using a moving window multi-taper Fourier transform (Chronux toolbox [Mitra and Bokil, 2008]) with a time-bandwidth product of five and seven tapers. The window size was 200 ms with 10 ms increments. In several figures, the gamma power was z-scored for display purposes (see figure legends). To determine whether and when an electrode responded selectively to conflict, we used a sliding F-statistic procedure (Liu et al. , 2009). Electrodes with differential responses between congruent and incongruent trials were selected by computing the F-statistic, for each time bin, comparing the neural responses between congruent and incongruent trials. Electrodes were denoted as ‘conflict selective’ if (1) the F-statistic exceeded a significance threshold for 50 consecutive milliseconds, and (2) the average neural response exceeded one standard deviation above the baseline period at least once during the trial. A null distribution generated by randomly permuting the labels was used to set the significance threshold with P = 0. 001. The latency at which congruent and incongruent stimuli could be discriminated was defined as the first time of this threshold crossing. For the response-aligned view, only electrodes where the latency preceded the response were included in subsequent analysis. This selection process was independently performed for each electrode in both stimulus-aligned and response-aligned analyses, and separately for the Stroop and Reading task. We used a permutation test with 10,000 shuffles to obtain a false discovery rate for our selection process. The congruent/incongruent trial labels were randomized 10,000 times and we measured the average number of electrodes across our population that passed the selection procedure. For the selected electrodes obtained with the procedure described in the previous section, we performed a number of within-electrode analyses. We measured single-trial correlations with behavioral reaction times, assessed the significance of interactions and simple/main effects, and controlled for confounds in measuring the neural Gratton effect. To account for both within-subject and across-subject variance, statistical testing of the electrophysiological data was conducted with multilevel models (Aarts et al. , 2014; Goldstein, 2011) (also known as random effect models). Random factors included electrodes nested within subjects. Significance of interactions and/or main effects was assessed with a likelihood ratio test against a null model excluding that particular term. For comparison of latency across regions, we restricted our analyses to simultaneous measurements made within each subject. We computed the latency difference for each pair of simultaneously recorded electrodes from different regions. The F-statistic of this latency difference across the groups was compared against a null distribution generated by shuffling, within each subject, the region labels (n = 10,000 shuffles). Post hoc testing used the Benjamin-Hochberg procedure to control for multiple comparisons. To measure cross-frequency coupling between the theta and gamma frequency bands, we used the Modulation Index (MI) defined previously (Tort et al. , 2008). Activity in the theta (4–8 Hz) and high gamma (70–120 Hz) bands was obtained with a zero-phase least-squares finite impulse response (FIR) filter. Instantaneous phase and amplitude was extracted with the Hilbert Transform. For the Stroop and Reading Task separately, the MI was computed as the Kullback-Leiber distance between the phase-amplitude histogram and a uniform distribution. For comparison between tasks, the number of trials was equalized. This MI was compared against a surrogate distribution generated by randomly lagging the time series across 1000 repetitions. Similar results were obtained with the measure defined in Canolty et al. (Canolty et al. , 2006). Results were also similar when a surrogate distribution was created by randomly pairing low-frequency phase with high-frequency power from different trials. To compare the strength of cross-frequency coupling between congruent and incongruent conditions, we computed the difference in MI between the two conditions while equalizing the trial count. This difference was compared against a null distribution generated by randomly shuffling the congruent and incongruent labels. | The brain adapts to control our behavior in different ways depending on the specific situation, which is particularly useful when deciding how to interpret conflicting sets of information. The' Stroop task' is a classic demonstration of this process. In this task, individuals are shown words where the color and the meaning of the text conflict – for example, the word' green' is written in blue. When asked what the color of the text is, individuals must suppress the instinct to read the word. This causes them to make more mistakes and take longer to decide on an answer than when they perform the same task using words that have no conflict (for example, when “red” is written in red). Previous work has suggested that several regions within part of the brain called the frontal cortex play a role in this cognitive control process. However, the relative contributions of each of these regions, and the order in which they are activated, remain unclear. This is in part due to the fact that accurately measuring the electrical activity of the frontal cortex requires implanting electrodes into the brain. Tang et al. took advantage of a rare opportunity to record this activity from a group of patients who had electrodes implanted in their frontal cortex to treat epilepsy. The electrical signals recorded by these electrodes as the subjects performed the Stroop task revealed that four regions in the frontal cortex altered their activity during trials where the color and the meaning of a word conflicted. These responses corresponded with the subject’s reaction time, changed depending on the exact nature of the task, and even reflected the subjects’ errors. These responses arose at different times in different regions, allowing Tang et al. to suggest how signals flow through the frontal cortex during cognitive control. In the future it will be important to further understand how the regions of the frontal cortex identified by Tang et al. interact with each other and to establish their roles in cognitive control. These observations could then be used to produce a theoretical framework that describes how the brain adapts behavior to different circumstances. | Abstract
Introduction
Results
Discussion
Materials and methods | neuroscience | 2016 | Cascade of neural processing orchestrates cognitive control in human frontal cortex | 12,187 | 436 |
"Myosin 5a is a dual-headed molecular motor that transports cargo along actin filaments. By followin(...TRUNCATED) | "Cells use motor proteins that to move organelles and other cargos from one place to another. The my(...TRUNCATED) | Abstract
Introduction
Results
Discussion
Materials and methods | structural biology and molecular biophysics | 2015 | "Structural dynamics of myosin 5 during processive motion revealed by interferometric scattering mic(...TRUNCATED) | 13,922 | 454 |
"The latent reservoir is a major barrier to HIV cure. As latently infected cells cannot be phenotype(...TRUNCATED) | "There is no cure for the human immunodeficiency virus infection (HIV), but anti-retroviral drugs al(...TRUNCATED) | Abstract
Introduction
Results
Discussion
Materials and methods | microbiology and infectious disease | 2020 | Phenotypic analysis of the unstimulated in vivo HIV CD4 T cell reservoir | 16,243 | 595 |
"Object manufacture in insects is typically inherited, and believed to be highly stereotyped. Optimi(...TRUNCATED) | "Male tree crickets produce sounds at a specific pitch to attract females. The louder the call, the (...TRUNCATED) | Abstract
Introduction
Results
Discussion
Materials and methods | ecology
computational and systems biology | 2017 | Tree crickets optimize the acoustics of baffles to exaggerate their mate-attraction signal | 6,671 | 569 |
"Missense mutations of valosin-containing protein (VCP) cause an autosomal dominant disease known as(...TRUNCATED) | "A disease called “inclusion body myopathy, Paget disease and frontotemporal dementia (IBMPFD) ”(...TRUNCATED) | Abstract
Introduction
Results
Discussion
Materials and methods | neuroscience | 2017 | "Valosin-containing protein (VCP/p97) inhibitors relieve Mitofusin-dependent mitochondrial defects d(...TRUNCATED) | 24,365 | 477 |
"Mammals produce volatile odours that convey different types of societal information. In Homo sapien(...TRUNCATED) | "Human body odour contains a number of chemicals, but the most pungent and recognisable are thioalco(...TRUNCATED) | Abstract
Introduction
Results
Discussion
Materials and methods | structural biology and molecular biophysics
microbiology and infectious disease | 2018 | Structural basis of malodour precursor transport in the human axilla | 14,273 | 385 |
"Monocytes are phagocytic effector cells in the blood and precursors of resident and inflammatory ti(...TRUNCATED) | "White blood cells form part of the immune system, which protects the body against infectious diseas(...TRUNCATED) | Abstract
Introduction
Results
Discussion
Materials and methods | cell biology
immunology and inflammation | 2015 | Immune surveillance of the lung by migrating tissue monocytes | 16,431 | 384 |
This is the "elife" split. For more words, refer to the PLOS split README
load with datasets:
from datasets import load_dataset
# If the dataset is gated/private, make sure you have run huggingface-cli login
dataset = load_dataset("pszemraj/scientific_lay_summarisation-elife-norm")
dataset
Output:
DatasetDict({
train: Dataset({
features: ['article', 'summary', 'section_headings', 'keywords', 'year', 'title', 'article_length', 'summary_length'],
num_rows: 4346
})
test: Dataset({
features: ['article', 'summary', 'section_headings', 'keywords', 'year', 'title', 'article_length', 'summary_length'],
num_rows: 241
})
validation: Dataset({
features: ['article', 'summary', 'section_headings', 'keywords', 'year', 'title', 'article_length', 'summary_length'],
num_rows: 241
})
})
Train set: